All published articles of this journal are available on ScienceDirect.
PLGA Polymers and Doxorubicin for the Treatment of Malignant Gliomas in Adults: An Overview
Abstract
Malignant brain tumors, such as glioblastoma and astrocytoma, are the most aggressive diffuse gliomas with a high grade (4) of malignancy in adults, leading to high mortality. The development of pharmacological approaches to drug delivery systems has led to increased effectiveness and reduced systemic toxicity of anticancer therapy. Delivery systems, such as implants, plates, gels, and micro- and nanoparticles, are used as carriers for anticancer substances, improving their solubility and biodistribution. These delivery systems affect the mechanism of drug action, reduce toxicity, Micro- and nanoparticles can penetrate physiological barriers of the body, including the blood-brain barrier (BBB). Due to changes in the microvascular system, they linger and accumulate in the area of pathology. Despite extensive data on delivery systems, only a few have undergone clinical trials and been adopted into clinical practice. For over 20 years, polymeric plates containing carmustine have been clinically used to treat malignant brain tumors. The search for safer and more effective forms of drug anti-tumor agents continues, as glioblastoma remains an incurable disease. Doxorubicin is a primary chemotherapy agent with proven efficacy, which is included in standard therapy for almost all tumor types. However, it is not used to treat central nervous system tumors, as it is believed that it does not cross the BBB. The polymers of lactic acid and glycolic acid (PLGA or PLG) are biocompatible and biodegradable. Standards for different tumor types where doxorubicin is used. We describe the mechanisms of action of polymeric and nanoparticle forms of chemotherapy drugs, the prospects of using PLGA polymers, and assess possible ways to deliver doxorubicin and other medications for brain tumors effectively.
1. INTRODUCTION
Doxorubicin is one of the most effective anti-tumor chemotherapy drugs used in the treatment of nearly all types of cancer [1], both in its standard form and with liposomal delivery systems. However, it is not used for brain tumors because it does not penetrate the BBB [2, 3]. Doxorubicin is administered by rapid intravenous infusion over 15-20 minutes. It has been found that cardiotoxicity decreases with prolonged infusion over 6 hours or more. A meta-analysis revealed that prolonged infusion reduces cardiotoxic effects with no differences in clinical response [4]. However, prolonged infusions may cause discomfort to the patient. The use of liposomal delivery may facilitate long-term drug administration. Moreover, the effectiveness of doxorubicin has been demonstrated in glioblastoma patients and human glioma cells in vitro [5]. Delivery systems based on PLGA polymers are capable of increasing the anti-tumor efficacy of drugs, such as doxorubicin [6], by avoiding peak concentrations and reducing toxicity. This is due to changes in pharmacokinetics [7, 8], increased solubility [9], the ability to penetrate the BBB, and accumulation in tumors due to the enhanced permeability and retention effect [10]. Liposomes with doxorubicin (Doxil®, Caelyx®, and Myocet®) have been approved by the FDA and are allowed for increased delivery to tumor tissues, while reducing accumulation in internal organs. However, their therapeutic efficacy increases only slightly [11, 12]. The half-life of liposomal doxorubicin is up to 72 hours compared to 10 minutes for the standard form [13]. Liposomal formulations are also associated with dose-dependent toxicity, which can lead to severe mucositis and hand-foot syndrome [14, 15]. The effectiveness of Gliadel® polymeric plates as a delivery system for the chemotherapeutic agent carmustine has been proven in the treatment of malignant brain tumors. The plates were placed in the cavity after tumor resection. In combination with temozolomide and radiation therapy, Gliadel® significantly improved the median survival duration of patients. Among different forms of delivery systems, biocompatible and biodegradable nanoparticles, including PLGA, are promising. PLGA is a lipophilic polymer that is approved by the FDA and European Medicines Agency and is used as a delivery vehicle for chemotherapeutics, anti-inflammatories, antibiotics, and other drugs. The complete biodegradability, simple synthesis, and controlled release of PLGA make it a promising delivery agent for these drugs. In experimental therapy of rats with glioblastoma, poloxamer-coated PLGA nanoparticles containing doxorubicin overcame the BBB, penetrated, and accumulated in tumors, thereby increasing the antitumor efficacy of doxorubicin [9]. The PLGA nanoparticle degrades into lactate and glycolate, which are metabolized in the Krebs cycle. The acid ratio determines the hydrophobicity and rate of degradation of PLGA [16]. However, one of the challenges of using PLGA for therapeutic purposes is low drug loading and rapid drug release from nanoparticles [7], which may be due to the predominant adsorption of the drug on the nanoparticle surface, leading to lower levels of drug reaching the target cells or tissues than expected after loading. According to the results of clinical studies, a recommended dose of PLGA nanoparticles containing doxorubicin with a satisfactory safety profile is 90 mg/m2 [17], while for liposomal doxorubicin (Caelyx®), it is only 50 mg / m2, and for the standard form of doxorubicin, i.e., 75 mg / m2. The use of delivery systems based on biodegradable polymers and the anti-tumor drug doxorubicin may be promising in the therapy of malignant gliomas. This work aimed to review research and clinical recommendations for the treatment of malignant brain tumors using doxorubicin and polymers. We used the PubMed and eLIBRARY.RU databases, as well as the Google Scholar search engine, to conduct a search for 70 guidelines based on evidence-based medicine for glioblastoma, astrocytoma, other types of brain tumors and tumors of other localizations from the National Comprehensive Cancer Network (NCCN®). The keywords used were: “glioblastoma,” “astrocytoma,” “doxorubicin,” “polymers,” “nanoparticles,” “PLGA,” “therapy,” and “toxicity”. The works reviewed were published between 1995 and 2024. A total of 218 literary sources were analyzed in preparing the review.
2. CHARACTERISTICS OF GLIOBLASTOMA AND ASTROCYTOMA: A STANDARD THERAPY FOR THEM
It is assumed that glioblastoma may arise from neural stem cells, neuroglial precursor cells, oligodendroglia and astrocytes, mesenchymal stroma cells, etc [18, 19]. Astrocytoma, on the other hand, arises from astrocytes [20]. The WHO revision of central nervous system (CNS) tumor classification in 2016 and 2021 changed the structure of glioma classification by incorporating molecular-genetic features of tumors in addition to histological features [21]. For glioblastoma, it was recommended to determine the IDH1/2 mutation status, which led to the separation of glioblastomas with IDH1/2 wild type (grade 4) and astrocytoma with mutant IDH1/2 (grade 4) in adults into separate diseases that belong to a general subgroup of diffuse high-grade gliomas in adults (Table 1). Pediatric-type diffuse gliomas were also systematized based on molecular genetic profiles, and key aberrations were included in their names. They are now no longer called “glioblastomas”. In diffuse IDH1/2 wild-type gliomas occurring in patient age groups younger than 65 (75-84) [18], the possibility of a patient having diffuse pediatric-type glioma should be considered [21]. The collective term “multiform glioblastoma” is no longer used [21].
Type of Tumor | Glioblastoma IDH1/2-wild-type | Astrocytoma IDH1/2-mutant/Refs |
---|---|---|
Source of origin | Develops de novo | Diffuse astrocytomas IDH1/2 mutants (grade 2-3) |
Incidence, % | ~ 90 | ~ 10 |
Median and peak age at diagnosis, years | 65(75-84) [22] | 38(40-50) [23] |
Localization | Supratentorial | Predominantly frontal |
Microvascular proliferation | +/- | +/- |
Necrosis | +/- | +/- |
Molecular-genetic characteristics | IDH1, IDH2 – wild-type; TERT promoter mutation; EGFR amplification/mutation; chromosome copy number changes: +7/-10 | IDH1 or IDH2 mutation; CDKN2A/B deletion; TP53 mutation; ATRX mutation |
Morphologically, IDH1/2 wild-type glioblastoma are highly cellular tumors that infiltrate brain parenchyma. Tumour cells are polymorphic with pronounced cell and nuclear atypia. The cellular composition may vary and include small, granular, multinuclear, giant cells, and gemistocytes. Different types of cells with pronounced histological diversity may be present in the same tumour. Mitotic activity is high. Necrosis in glioblastomas often has a pseudopalisading type [24]. However, the histopathological criteria for necrosis and vascular proliferation in the classification of high-grade diffuse gliomas have lost their prognostic value, and prognostic markers have now been identified as IDH1/2 mutations and homozygous deletions of CDKN2A/B [25]. Additionally, these tumors are characterized by point mutations in the TERT promoter region (74-83%), homozygosity deletion of CDKN2A (37-58%), and amplification/mutation of EGFR (25-50%). These genetic features correlate with a negative prognosis for glioblastoma [24]. The methylation status of the MGMT promoter is one of the prognostic biomarkers for this type of cancer. With MGMT gene methylation, the response to alkylating agents, such as temozolomide, is better. The frequency of TP53 mutations is lower in glioblastomas (25-37%) compared to IDH1/2 mutant astrocytoma (about 80%). Mutations in ATRX are rare in glioblastoma with wild-type IDH1 and IDH2 but common in astrocytoma with IDH1 or IDH2 mutations [24].
Morphologically, cases of IDH1/2 mutant astrocytoma are indistinguishable from wild-type IDH1/2 glioblastoma. The classification of CNS tumors is no longer strictly histological, as the presence of a homozygous deletion in CDKN2A or CDKN2B classifies a tumor as grade 4, the highest grade of malignancy, even if there is no microvascular proliferation or necrosis. Therefore, glioblastomas should be diagnosed in diffuse astrocytic gliomas with IDH1 or IDH2 wild type in adults if they have microvascular proliferation, necrosis, TERT promoter mutation, EGFR gene amplification, or changes in the number of chromosomes (+7/-10) [24]. For IDH1/2 mutant
Type of Tumor/Therapy Method | Glioblastoma IDH1/2 wild type | Astrocytoma IDH1/2 mutant | Advantages of the Method/Refs | Disadvantages of the Method/Refs |
---|---|---|---|---|
Surgical resection | Subtotal/Total | High survival rate with 90% tumor resection, biopsy (diagnosis), palliative care | The low survival rate with less than 90% tumor resection, post-operative edema, infection, risk of metastasis outside the CNS up to 2% | |
Laser interstitial thermotherapy | - | Minimally invasive method in patients with inoperable craniotomy [26, 27] | Additional research needed; for transient aphasia, temporary hemiparesis, and dysphagia [28] | |
Radiotherapy | 3D, targeted, hypofractionated [29] | Reactivation of the anti-tumor immune response [31] | Nausea, vomiting, oral ulceration, alopecia, skin pigmentation [32]; development of resistance [33]; risk of severe myelotoxicity [34]; development of myelodysplastic syndrome [35]; decrease in hematopoiesis, especially granulocyte and thrombopoiesis; cardiotoxicity; nephrotoxicity – glomerular atrophy and increased permeability of glomerular capillary endothelium [36]; progression [38] Patients receiving carmustine wafers are at higher risk of seizures and postoperative infections [40] Cardiovascular toxicity [41], gastrointestinal perforation, pancreatitis, arterial thromboembolism, hypertension, palmarplantar syndrome [42], toxicity to the nervous system [43], neutropenia, infections, nephrotic syndrome, liver damage, bleeding, thrombocytopenia, anemia, leukopenia, visual impairment, diarrhea, nausea, vomiting, stomatitis, malignant basal cell tumor, cutaneous squamous cell carcinoma, keratoacanthoma, melanoma, dermatitis, pneumonitis, rhabdomyolysis, facial nerve paralysis, arthritis, fever, peripheral edema, asthenia, hypersensitivity reactions, progression of previous chronic myelomonocytic leukemia, pancreatic adenocarcinoma, suppression of bone marrow function and pulmonary fibrosis [49-51], pseudo-response, and progression [52] |
|
Chemotherapy (Local/Systemic) At recurrence or disease progression If the preferred or other recommended regimens are ineffective or intolerable |
Temozolomide; polymer wafers with carmustine (BCNU) in the resection cavity [30] | Increase in radiosensitivity [39] Increase in survival, palliative care Increase in survival, overcoming resistance |
||
Combination of lomustine and temozolomide [37] | - | |||
Temozolomide, a combination of procarbazine, carmustine/lomustine, and vincristine, polymer wafers (implants) with carmustine (BCNU) in the resection cavity Etoposide, platinum drugs |
||||
Antibodies/Inhibitors (Systemic) | Bevacizumab, regorafenib (VEGF A; VEGFR); Tumors with NTRK gene fusion (Larotrectinib; Entrectinib); BRAF/MEK inhibitors (For BRAF V600E Mutation): Dabrafenib/trametinib; Vemurafenib/cobimetinib |
Ivosidenib [44] | Increase in radiosensitivity [45] and chemosensitivity [46] Increase in survival [47], overcoming resistance |
|
Tumor-treating fields therapy | - | Increase in survival, palliative care [48] | Inconvenience of use; additional studies on effectiveness needed [53] |
Drug | Drug Formulation/ Route of Administration | Tumor Type |
---|---|---|
Doxorubicin | Standard form (Systemic) |
Malignant tumors: bladder, breast HER2+/-, cervical, salivary glands, kidney [64], Merkel cell carcinoma, neuroendocrine, adrenal, prostate, colorectal, small cell lung [65], thymus, thyroid [66], endometrial, uterine [67]; Richter's transformation in Hodgkin's lymphoma [68]; mesothelioma, peritoneal, multiple myeloma, neuroblastoma, intermediate-risk nonseminomas, Wilms' tumor (nephroblastoma); cholangiocarcinoma [69]; macroglobulin anemia; soft tissue sarcomas; lymphomas/leukemias: Burkitt's, marginal zone, classic follicular, B-cell, including pediatric, HIV-associated, mantle cell, diffuse large B-cell, post-transplant, Waldenström's/macroglobulinemia, Hodgkin's, including pediatric, anaplastic large cell associated with breast implant [70], T-cell [71], hepatosplenic; pediatric and adult acute lymphoblastic, hairy cell; blastic plasmacytoid dendritic cell neoplasms, vaginal cancer |
Liposomes (Systemic) [72] |
Malignant tumors: invasive breast, ovarian, fallopian tube, peritoneal, uterine, endometrial, cervical; mycosis fungoides/Sézary syndrome; lymphomas/leukemia: primary cutaneous, adult T-cell [73], Castleman's disease, Hodgkin's [74, 75]; Kaposi's sarcoma [76], multiple myeloma [77], soft tissue sarcomas [78], prostate [79] | |
Microspheres (Local/Chemoembolization) |
Uveal melanoma, liver metastases [80]; cholangiocarcinoma, hepatocellular carcinoma [24, 81-83] | |
Daunorubicin | Liposomes (Systemic) |
Chronic lymphocytic leukemia/small lymphocytic lymphoma histologic transformation (Richter) [84]; Kaposi sarcoma [76]. |
Vincristine | Liposomes (Systemic) |
Uveal melanoma [85] |
Irinotecan | Liposomes (Systemic) |
Malignant tumors of the biliary tract [86, 24]; adenocarcinoma of the pancreas [87, 88] |
Carmustine | Biodegradable polymeric wafers (Local) | Glioblastoma IDH1/2 wild-type and astrocytoma IDH1/2 mutant, grade 4 [89, 90] |
Aspargase | PEGylated form of engineered protein | Acute lymphoblastic leukemia, pediatric acute lymphoblastic leukemia, extranodal NK/T-cell lymphomas [91-93] |
Paclitaxel | Albumin nanoparticles | Breast cancer, non-small cell lung cancer, pancreatic cancer, melanoma, biliary tract cancers [94-98] |
Interferon alpha subtype 2b | PEGylated form | Polycythemia vera, adult T-cell leukemia/lymphoma, systemic mastocytosis, mycosis fungoides/sezary syndrome, melanoma, B-cell lymphomas, bone cancer, histiocytic neoplasms [99-107] |
Cytarabine and daunorubicin | Liposomes | Acute myeloid leukemia [108] |
Rapamycin/sirolimus | Albumin nanoparticles | Perivascular epithelioid cell tumors, soft tissue sarcoma [109, 110] |
Lanreotide acetate | Microparticle or Autogel | Gastroenteropancreatic neuroendocrine tumors |
Cytosine arabinoside | Liposomes (CSF) | Leptomeningeal metastases (lymphoma, breast cancer), acute lymphoblastic leukemia [111-113] |
Filgrastim | PEGylated form (Subcutaneous) | Bladder cancer, gestational trophoblastic neoplasia, hairy cell leukemia [114, 115] |
astrocytoma compared to IDH1/2 wild-type cases, there is an increased frequency of MGMT methylation, a decreased frequency of TERT mutations (5-28%), and no EGFR amplification or PTEN mutation [24].
The genome and epigenome of glioblastomas are well described, and biological subtypes have been identified. These subtypes are defined by their gene expression profiles: proneural, classical, and mesenchymal. Although this has not influenced patient survival or the treatment approach, it is likely due to intratumoral heterogeneity in glioblastomas [18]. Despite significant molecular genetic research on malignant gliomas, which has improved the classification of CNS tumors and increased treatment efficacy and prognosis, long-term patient survival remains low [24]. Standard therapy for patients with diffuse malignant glioma includes surgical resection, radiation therapy, chemotherapy (temozolomide), and alternating electric current therapy (Table 2). However, these treatments do not have a long-lasting anti-tumor effect [25]. Without treatment, the median overall survival for patients with glioblastoma from the time of diagnosis is no more than 3-4 months. After standard therapy, it can be up to 13-16 months [25]. One of the main mechanisms of anti-tumor activity of chemotherapeutics is the inhibition of DNA and protein synthesis, as well as alkylation, methylation, and DNA damage caused by free radicals [191].
The median overall survival of patients with IDH1/2-mutant astrocytoma after treatment is higher and amounts to 54 months [25]. Total resection of glioma, regardless of the molecular status of the tumor, increases life expectancy for patients [23], but due to the diffuse growth of the tumor, complete resection is not always possible. The treatment of malignant diffuse gliomas is also complicated by the heterogeneity of genetic drivers [54] and innate or acquired resistance of tumor cells to known therapy [55, 56], which also have pronounced toxic side effects [57, 58].
Thus, malignant diffuse gliomas in adults are currently incurable. The modern standard of therapy helps to prolong life and alleviate the symptoms of the disease, but it does not affect long-term survival. Therefore, the search for more effective treatment methods continues.
3. DOXORUBICIN, AS A PART OF THE MODERN STANDARD THERAPY FOR ONCOLOGICAL DISEASES: MECHANISMS OF ITS ANTITUMOR AND TOXIC EFFECTS
Doxorubicin is one of the main drugs included in the standard therapy for most types of tumors (Table 3). In accordance with the treatment protocol, doxorubicin can be administered systemically as a single agent or in combination with other drugs, either in standard form or as liposomes, both for initial treatment and for recurrent or progressive cancers that have developed resistance to standard therapies, as well as for supportive and palliative care [1]. Doxorubicin is part of a group of essential medications available in the form of concentrate for preparing solutions for infusion, intra-arterial administration, and intravenous injection. Despite its strong antitumor activity, the low permeability of doxorubicin through the BBB has limited its use in tumors of the central nervous system [2, 3].
The mechanisms of toxic and anti-tumor action of doxorubicin are complex and not fully understood. Doxorubicin inhibits topoisomerase II [59], which is associated with its anti-tumor activity. Its toxic effects are also associated with the formation of reactive oxygen species and their impact on cell membranes and organelles [60, 61]. The high affinity of doxorubicin for cardiolipin enables it to accumulate in mitochondria, leading to mitochondrial dysfunction due to oxidative stress [62].
4. POLYMERS AND NANOPARTICLES USED IN MODERN STANDARDS OF THERAPY FOR CANCER: MECHANISMS OF THEIR ANTITUMOR ACTION
Despite numerous preclinical and clinical studies on different delivery systems, such as devices, gels, plates, films, micro- and nanoparticles, which have been conducted since 1958 [63], only a few of them have entered the modern standards for the treatment of some types of cancer (Table 3).
Polymer plates with carmustine (1,3-bis(2-chloroethyl)-1-nitrosourea (BCNU), better known as Gliadel® plates, are a part of the evidence-based standard of therapy for malignant gliomas, glioblastoma and astrocytoma [1]. The plates consist of a polyanhydride polymer, which is formed by the polymerization of a carboxylic acid with anhydride bonds and is the most studied, proven biocompatible, and biodegradable polymer for tumor therapy [89]. The plates are biodegradable implants approved by the FDA in 1996 for patients with recurrent glioblastomas as an adjunct surgery. Gliadel® plates were the first FDA-approved method for conducting local prolonged-action chemotherapy for brain tumors. This significantly improved the median survival of patients [89]. In a 2022 observational study on 506 patients with malignant glioma who received adjuvant treatment with Gliadel® plates, an increase in median overall survival to 18 months was found. Of these, 39.8% of patients survived for two years and 31.5% for three years. These encouraging results open up possibilities for the development of new approaches to treating malignant brain tumors using biocompatible polymers as drug carriers, including nanoparticles [90].
One of the main tasks in the development of new polymeric materials is to achieve controlled and prolonged delivery of drugs to brain tumors. Polymeric nanoparticles, nanofibers, and gels allow for an increased residence time of drugs in the tumor, which contributes to their increased effectiveness. In immunotherapy, PLGA nanoparticles can protect antigens from degradation, increase the likelihood that they will be presented to immune cells, and reduce side toxic effects [116].
During tumor growth, morphological and molecular changes occur in tumor tissues. These changes have divergent effects; some contribute to the efficacy of therapy with micro- and nano-sized forms of drugs, such as the EPR (enhanced permeability and retention) effect, hemorrhages, edema, and microleaks from vessels, all of which contribute to systemic therapy. However, other changes hinder treatment. Due to the heterogeneous BBB during tumor growth [117], thromboses [118], and impaired lymphatic drainage, which contributes to edema and increased intracranial pressure [119, 120], many drugs may poorly penetrate tumor tissues, and their concentrations may be insufficient for a pronounced antitumor response. It has been shown that only about 20% of temozolomide, a first-line drug used in the treatment of glioblastoma and grade 4 astrocytoma, penetrates tumors when it is introduced into the systemic circulation [121].
Upon intravenous injection of nanoparticles, circulating serum proteins attach to the surface of the nanoparticles, forming a “protein corona” [122]. The antitumor action of PLGA nanoparticles with doxorubicin may be facilitated by the adsorption of apolipoprotein E (APO-E) on the surface of nanoparticles, followed by their interaction with APO-E receptors on the surface of endothelial cells in brain vessels, overcoming the BBB due to extravasation through receptor-mediated transcytosis [123, 124]. These effects, along with the “passive” extravasation of nanoparticles caused by tumor-specific leakage from blood capillaries with incompletely formed walls and ineffective lymphatic drainage in tumors (EPR effect), contribute to the accumulation of nanoparticles in tumor tissues [123, 124].
It is known that lactate receptors are overexpressed in glioblastoma cells [125]. It has been found that PLGA nanoparticles enter glioma cells through clathrin-dependent endocytosis [126]. The pH inside PLGA nanoparticles can reach 2 [127]. The acidic microenvironment can affect the effectiveness of the administered drug. The formation of acids during the degradation of PLGA may destabilize acid-sensitive drugs contained in the nanoparticles and reduce their efficacy [90]. However, some data suggest that acidification within a tumor cell can increase the antitumor efficacy of chemotherapeutic drugs, such as doxorubicin [128, 129] and paclitaxel [128]. Under conditions of high acidity (pH < 4) or high alkalinity (pH > 11), active oxygen species and enzymes, such as hyaluronidases, destroy the intercellular matrix of tumor tissues and areas of invasive tumor growth more efficiently. This promotes the release of antitumor substances in these areas [147]. Alkaline intracellular pH (pHi) promotes DNA synthesis and tumor cell proliferation, increasing their number and tumor volume [129]. Also, the existing pHi threshold, around 7.1-7.2, is the limit below which enzymes lose their activity, and growth factors cannot stimulate the progression of the G1 phase of cell division and entry into the cell cycle [130]. It has been shown that the activity and expression of the Na+/H+ antiporters, involved in maintaining pHi, increases in doxorubicin-resistant tumor cells, and a decrease in pHi in the cell contributes to the intracellular accumulation of doxorubicin, reducing its level of resistance [128]. It has been noted that pHi in drug-resistant cells is higher, and an acidic environment increases DNA binding with the alkylating agents, such as cisplatin, which increases its antitumor efficacy. The use of bafilomycin, a hydrogen pump inhibitor, contributes to the reduction in pH of tumor cells, increasing the cytotoxicity of cisplatin. An increase in pH promotes the transition of tumor cells to G2/M [131-148], thus stimulating cell proliferation. It is assumed that a decrease in pHi is an early signal for the activation of caspases during apoptosis. Cytochrome-mediated caspase activation requires cytosolic acidification, with the highest caspase activity at a pHi of 6.3-6.8 [149]. With an increase in alkaline pHi, the activity of glycolysis increases, which is necessary for energy production in rapidly growing tumor cells [150, 151].
One of the mechanisms of action of polymers in the treatment of brain tumors is the enhancement of the effect of radiotherapy. Radiosensitive polymers can exacerbate tumor cell damage, which can be used in glioblastoma using surgical access [152].
Thus, the formation of acids as a result of the degradation of PLGA can contribute to the antitumor effect both by increasing the antitumor activity of doxorubicin and by stopping the cell cycle, inhibiting the activity of the enzyme system and ion channels of tumor cells. Whereas, due to increased expression of lactate receptors on tumor cells, PLGA nanoparticles can selectively accumulate in them.
PLGA nanoparticles, upon intravenous administration, predominantly accumulate in the liver and spleen. Capture and absorption of nanoparticles by macrophages occur after their opsonization by Fc fragments of immunoglobulins. Surface modification of particles reduces the capture opsonization of nanoparticles by macrophages in the liver, spleen, and other tissues, increase the effectiveness of targeted delivery of nanoparticles to tumor cells [152, 153].
The kidneys play an important role in the clearance of nanoparticles. Their excretion depends on the size, shape, surface modification, and charge. Individual studies have reported that both an increase in the accumulation of polyethylene glycol-coated nanoparticles in the kidneys [154-156] and the prevention of their accumulation [157, 158] can occur. There is also evidence that the polyethylene glycol coating does not affect the clearance of these nanoparticles [159, 160]. Presumably, this is due to the fact that these coated nanoparticles are less likely to be captured by cells in the reticuloendothelial system. The size and charge of the nanoparticles are important factors in determining their pharmacokinetics and distribution in the body. Particles smaller than 6 nm undergo rapid renal clearance, while particles larger than 200 nm are metabolized in the liver and spleen. The half-life of nanoparticles and macromolecules in the blood and body increases with their diameter [161, 162]. However, there have been reports on the detection of nanoparticles in urine in an unchanged form after injection into laboratory mice [122]. Nanoparticles can enter through the outflow renal arteriole (Arteriola glomerularis efferens) into the capillaries surrounding the tubules of the nephron, enter its lumen, and then are excreted in the urine.
Systemically administered nanoparticles with a diameter of <5 nm are excreted through renal filtration. Larger nanoparticles (> 200 nm in diameter) cannot effectively reach the BBB due to sequestration in the spleen. PLGA nanoparticles coated with polyethylene glycol, with a size of 100 nm, have a longer circulation time and better accumulation in brain parenchyma compared to particles with sizes of 200 nm and 800 nm for mice with traumatic brain injury. However, Nowak et al. (2020) found that polystyrene nanoparticles with a diameter of 200 nm penetrate the BBB more effectively than those with diameters of 100 nm and 500 nm. Other studies reported that the size of the nanoparticles has a limited impact or does not affect their ability to penetrate the BBB at all. These contradictory results highlight the challenges in developing treatments for brain tumors, as the BBB, extracellular space, and physiology differ significantly between in vivo and in vitro models and humans.
5. CHARACTERISTICS OF PLGA-BASED POLYMERIC DELIVERY SYSTEMS AND THERAPEUTIC PROSPECTS FOR THEIR APPLICATION
Due to the distinctive characteristics of PLGA polymers, they have significant potential for brain tumor treatments. These polymers are completely biodegradable and exhibit mechanical stability [163]. They are relatively straightforward to produce and widely accessible commercially. They ensure stable drug release and offer modifiable properties. They are also biocompatible [90]. The PLGA polymer is used in various medical devices, such as screws, plates, stents, wound dressings, and tissue adhesives. It is also used as a material for surgical meshes, drug carriers, and tissue engineering (Fig. 1) [63]. Depending on the required effect, the degradation rate can be changed from a few days to a few years. RESOMER® is the most commonly used brand of PLGA.
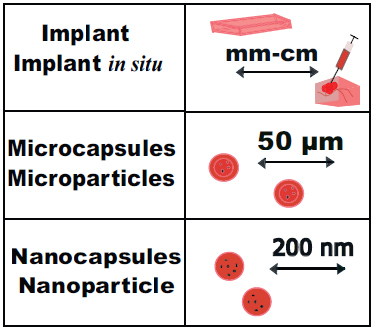
In the body, polymer degradation occurs due to hydrolysis and the action of the esterase enzyme [165]. PLGA degradation is heterogeneous; it occurs faster in the central part of the delivery system. When water is present, PLGA undergoes hydrolysis, where water first wets the surface and then diffuses into the polymer. Hydrolysis of the ester bond then occurs, splitting the polymer chain into shorter chains that gradually dissolve, leading to erosion of the polymer. The resulting monomers and oligomers are excreted by the kidneys or metabolized into carbon dioxide and water [16]. PLGA polymers are negatively charged, limiting their cellular uptake via negatively charged cell membranes. It is assumed that the specific targeting unit for PLGA nanoparticles and other particles are all pathological conditions of the body associated with damage to the vascular wall.
Peptide-loaded PLGA implants are widely used as drug carriers with a duration of action from 1 to 6 months for the treatment of hormone-sensitive malignant tumors of the prostate or breast [166]. The implant is administered subcutaneously or intramuscularly, and the drug is released in a controlled manner over an extended period of time. Small-sized intravitreal PLGA implants loaded with dexamethasone are used to treat macular edema of the eye [167]. One approach is the formation of in situ implants. For this, the PLGA polymer PLGA is dissolved in a biocompatible organic solvent and administered to the patient. This is easier to use, requires less expense, and uses smaller needles. PLGA microspheres (1 µm to 1000 µm micrometers in size) are typically used for parenteral delivery of hydrophilic or hydrophobic drugs. Inside the microsphere, drugs are either solubilized or dispersed. The release profile depends on the size distribution and porosity of microspheres, the characteristics and concentration of drugs, and release conditions. There are several phases in the drug release profile: initially rapid release followed by a slower phase, and finally, an accelerated release phase. This accelerated final release is caused by acidic pH inside particles due to the autocatalytic degradation of polymers [168]. Nanoparticles and nanocapsules with sizes between 20 and 200 nanometers can be made from PLGA polymers. The main mode of administration for these nanosized delivery systems is through intravenous injections, but other routes, such as oral, dermal, pulmonary, or ocular administration, are also possible [16]. Thus, PLGA polymer in the form of microspheres is used intramuscularly and subcutaneously with leuprolide acetate (Eligard®, Leuprolide acetate®, Durin®) for prostate tumors and Alzheimer's disease [169]. Subcutaneously, octreotide acetate is administered to patients with acromegaly and oncological patients with intestinal tumors and neuroendocrine carcinoid tumors. Microspheres containing goserelin acetate are used for hormone-sensitive breast and prostate tumors [170]. Gels containing doxycycline hyclate are used to treat periodontitis and cancer pain. An implant-pump is used for prolonged intrathecal infusion of ziconotide acetate for cancer treatment [166, 171]. PLGA Trelstar LA microspheres with the hormone triptorelin pamoate are used to treat breast and prostatic tumors [172]. PLGA gel Oncogel® with paclitaxel is used in anticancer therapy [173].
In glioblastoma, inhibition of AKT/mTORC1/GPRS and PI3K/AKT signaling pathways, targeting the glioma microenvironment, and modifying PLGA nanoparticles will be attempted. It is possible that the complex targeting of the main targets is related to glioma, including activation of the growth factor pathway, cell destruction, cycle checkpoints that control cell division, and abnormal telomere maintenance, which may improve patient survival and stimulate the immune system to treat glioblastoma. PLGA-temozolomide and IL-15 nanoparticles with NK cell membrane-decorated try to elicit the immunostimulatory microenvironment for glioblastoma chemo-immunotherapy.
6. PRECLINICAL AND CLINICAL STUDIES ON DRUG DELIVERY SYSTEMS USING POLYMERS AND DOXORUBICIN
The main polymers used in the development of drug delivery systems for tumor therapy include doxorubicin, which is divided into natural and synthetic categories. Natural polymers, such as gelatin, albumin, collagen, alginate, hyaluronic acid, dextran, cellulose, chitosan, mannan, and pullulan, and synthetic polymers, such as polyglycolic acid, polyglutamic acid, polylactide, poly(lactide-co-glycolide) and poly(lactic acid) as well as polyvinyl alcohol, polylysine and pluronic are also used for developing new anticancer drugs (Fig. 2).
Due to their unique characteristics, PLGA polymers have great potential for brain tumor treatment. They are exhibit mechanical stability. They are relatively easy to produce and widely available commercially. They ensure stable drug release and offer modifiable properties.
The use of existing, well-studied drugs, which are used for other types of tumors, is one of the alternatives in research. The development of new drugs often takes a long time and costs a lot of money. Most new molecules fail to be effective. Drug repurposing, with proven efficacy, is possible through the use of new forms of drugs, for example as part of delivery systems. Repurposing existing drugs is also cheaper and faster than developing new ones [174].
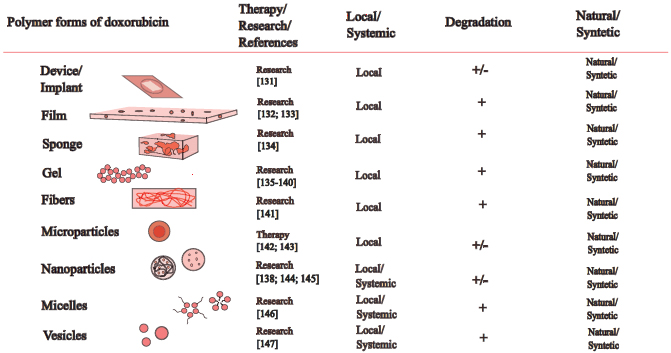
Polymeric forms of doxorubicin used in the clinic and preclinical/clinical trials in oncology.
Form | Route of Administration | References |
---|---|---|
Preclinical trials | ||
Nanoparticle | Systemic | [6, 10] |
Nanoparticle | Intranasal | [189] |
Nanoparticle/theranostics | Systemic | [190] |
Nanoparticle/ multicomponent/modification | Systemic | [191] |
Scaffold/ multicomponent/modification | Systemic | [192] |
Nanoparticle/ multicomponent | Systemic | [193] |
Clinical trial | ||
Nanoparticle | Systemic | [17] |
Maksimenko O. et al. (2019) and Kudelkina V. et al. (2021) used PLGA nanoparticles with doxorubicin to treat rat glioblastoma 101.8 and found that the nanoparticles penetrate through the BBB into intracranial tumors, increasing the antitumor efficacy of doxorubicin in vivo (Table 4).
Anti-tumor effects in vitro were also observed against two human glioma cell lines using PLGA-loaded nanoparticles containing the chemotherapeutic agent morusin and conjugated to chlorotoxin, a peptide specific for certain chloride channels expressed on glioma cells. These effects were statistically significant [175]. Similar results were also obtained in vitro with PLGA nanoparticles [176-178] and in vivo with rodent models of brain tumors [178], opening up the possibility of their clinical application [90]. To improve the delivery of carboplatin as a chemotherapeutic, PLGA conjugates with folic acid receptors, overexpressed on tumor cell membranes, including those of glioblastomas, were developed [179].
To increase the accumulation of anti-tumor drugs in the tumor, a method of convection drug delivery under pressure is used. This method is introduced through a catheter placed in glioblastoma tissues. This leads to an increased volume of distribution compared to diffusion, allowing drugs to spread through brain tissues more efficiently [180]. The effectiveness of doxorubicin has been found in humans with intratumoral administration [181, 5]. Focused ultrasound can increase the permeability of the BBB and penetration of drugs into tumor tissue [90]. Nanoparticles can also be delivered into the brain tissue using phagocytic cells, such as monocytes, neutrophils, and stem cells. Immune cells like monocytes and neutrophils are particularly attractive vectors for nanoparticle delivery because they easily penetrate the BBB to sites of injury, inflammation, and tumors [90]. Clinical trials of PLGA membranes with anti-tumor substances are currently underway [182]. After the surgical removal of soft tissue tumors, PLGA membranes containing drugs are placed in the surgical cavity. In clinical studies on malignant esophageal tumors, nanoparticles of PLGA [183] were used as carriers for an immunomodulating agent consisting of trehalose-6, 6-dimycolate, an invariant activator of natural killer T cells. An oral form of the PLGA tablet [184] was used as a vaccine for patients with melanoma containing tumor proteins and granulocyte-macrophage colony-stimulating factor, oligodeoxynucleotide, and lysates. PLGA nanoparticles protect antigens from degradation, increase their presentation to immune cells, and reduce toxic side effects [116]. Thus, the implant Ozurdex™, containing dexamethasone, slowly decomposes in the polymer PLGA delivery system Novadur® [185], releasing the drug. Currently, this medicinal form of dexamethasone is undergoing clinical trials for its efficacy in patients with retinal detachment due to uveal melanoma.
In addition to its anti-tumor activity, a reduction in drug toxicity in nanoparticles has been demonstrated. Thus, statistically significant reductions in hematological toxicities, as well as normal levels of erythrocytes, platelets, and hemoglobin, along with cardiotoxicity and testicular toxicity from doxorubicin, were observed in PLGA nanoparticle formulations [186]. Nephrotoxicity was also described in another study [187].
Targeting polymeric drug delivery systems that take advantage of the overexpression of specific molecules and proteins in tumor cells, as well as the microenvironment and extracellular components of the intercellular matrix, may be more effective for anti-cancer therapy [188-204]. Antitumor effectiveness can be increased by targeting multiple targets at once. Compared with single-ligand targeting of PLGA-docetaxel to human U87-MG glioblastoma cells, targeting two ligands at once, the EGFR and PD-L1, is more effective [199].
PLGA nanoparticles are targeted with folic or hyaluronic acid, transferrin for breast cancer treatment [200, 202] and glioblastoma [206], conjugated with antibodies to neuronal cell adhesion molecules in lung cancer [201], and used for targeting artesunate and chloroquine to colorectal cancer [198]. PLGA nanoparticles modified with PEG, biotin, or EpCAM aptamer were used to enhance cellular uptake and cytotoxicity of doxorubicin in breast cancer, non-small cell lung cancer, and prostate cancer [203, 205].
Chemotherapy for malignant gliomas is often given systemically, either through intravenous or oral routes. However, the toxicity of these treatments limits the dose that can be administered. To reduce this toxicity and improve the targeted delivery of antitumor drugs, researchers are exploring alternative routes of administration, such as intraarterial and intranasal routes, which limit systemic toxicity [206-209].
Intranasal administration of polymeric nanoparticles loaded with antitumor drugs into the brain is a non-invasive and painless method that helps to bypass the BBB, increasing the bioavailability and effectiveness of therapy due to enhanced penetration and residence time of the drug in the brain. This approach has been shown to enhance the anti-angiogenic effects of bevacizumab in the treatment of glioblastoma [210].
Intranasal-administered nanoparticles allow for rapid absorption, reduced systemic toxicity, decreased enzymatic degradation, and increased bioavailability of the active agent, leading to a more prolonged and effective pharmacological response. This method allows drug delivery from the nasal cavity directly to the brain through the trigeminal and olfactory nerves, but it is limited by the small surface area of exposure, low epithelial permeability, and the small volume of drug that can be delivered. Additionally, the drug can be removed from the absorption site by nasal secretions [211, 212].
Intracerebroventricular infusion and convection-enhanced delivery are effective treatment options for brain tumors, including using doxorubicin. However, these methods are invasive and require precise injections into the tumor [213].
Additionally, in clinical trials, PLGA implants loaded with high-dose carmustine have been placed into the cavity after glioblastoma resection in patients. This treatment was evaluated and showed good safety results [214].
Moreover, an alternative direct method for drug delivery to the brain could be convection-enhanced delivery, which does not depend on diffusion and allows for precise control of the infusion rate. In this method, drugs are evenly distributed over a large area of the brain, regardless of the size of the molecule administered. However, due to the absence of specific drug targeting, neurotoxicity may occur [215].
CONCLUSION
Glioblastoma and astrocytoma (grade 4) are two rare but deadly brain tumors that affect adults. Unfortunately, there is no cure for them, and the main driver mutations that could potentially be targeted in clinical practice have not been identified in most cases. A total of 9,386 mutations have been detected, including 199 genes that affect patient survival [194]. Standard systemic and local chemotherapy for glioblastoma and astrocytoma has increased the lifespan of patients, but long-term survival of up to 5 years remains extremely low. Tumor cells are resistant to anti-tumor treatments, and the BBB prevents the accumulation of many drugs. Moreover, no driver mutations have been found in glioblastoma or astrocytoma, whose signaling pathways can be targeted. Currently, the treatment of glioblastoma patients is mainly focused on palliative care and therapies that increase life expectancy. Despite this, glioblastomas recur in almost 100% of cases. Therefore, research on new anti-cancer substances and methods for treating glioblastomas continues.
The use of doxorubicin for brain tumors is justified due to its high anti-tumor activity in most types of malignant tumors. Different polymeric forms of doxorubicin, methods of administration, and their combinations can help to overcome the BBB and resistance, increase the anti-tumor efficacy, and reduce toxicity. Conjugation or encapsulation of micro and nanoparticles in PLGA improves the delivery of doxorubicin to malignant gliomas and increases its antitumor activity. This can occur due to the selective accumulation of particles in tumor tissues, the EPR effect, acidification of the extra- and intracellular environment of the tumor, and the acidic composition of the PLGA polymer.
Safe, biocompatible polymers are promising materials for the development of delivery systems for local and systemic administration, such as implantable devices, plates, gels, and nanoparticles for controlled and prolonged targeted delivery of anti-tumor drugs, allowing them to overcome BBB and drug resistance. The development of local therapy using postoperative access is especially relevant because almost all patients undergo surgical treatment. Polymeric carriers are used locally, with plates that actively release drugs directly into the tumor growth site placed in the surgical bed. Combining local and systemic drug delivery may be an effective strategy in fighting the tumor. PLGA is a promising polymer for delivering anti-tumor agents because it completely decomposes into water and carbon dioxide, contributing to increasing effectiveness and reducing toxicity of anticancer drugs.
AUTHORS’ CONTRIBUTION
It is hereby acknowledged that all authors have accepted responsibility for the manuscript's content and consented to its submission. They have meticulously reviewed all results and unanimously approved the final version of the manuscript.
LIST OF ABBREVIATIONS
APO-E | = Apolipoprotein E |
ATRX | = Alpha-thalassemia mental retardation syndrome |
BBB | = The blood–brain barrier |
BCNU | = 1,3-Bis(2-chloroethyl)-1-nitrosourea |
CDKN2A | = Cyclin-dependent kinase inhibitor 2A |
CNS | = The central nervous system |
EGFR | = The epidermal growth factor receptor |
EpCAM | = Epithelial cell adhesion molecule |
Fc | = Fragment crystallizable |
IDH | = The isocitrate dehydrogenase |
MEK | = Mitogen-activated protein kinase |
MGMT | = Methylguanine methyltransferase |
NCCN | = The national comprehensive cancer network |
NK | = Natural killer |
NTRK | = Neurotrophic receptor tyrosine kinase |
PD-L1 | = Programmed death-ligand 1 |
PEG | = Polyethylene glycol |
pHi | = Intracellular pH |
PTEN | = Phosphatase and tensin homolog |
VEGFR | = Vascular endothelial growth factor |
FUNDING
This study is financially supported by the Ministry of Science and Higher Education of the Russian Federation within the framework of Agreement No. 123030700107-4, the cellular and molecular mechanisms of tumor progression.
ACKNOWLEDGEMENTS
The research was conducted using the scientific infrastructure 'Collection of Experimental Tumors of the Nervous System and Neural Tumor Cell Lines', supervised by Dr. A.M. Kosyreva.