All published articles of this journal are available on ScienceDirect.
Molecular Insights into Oxidative Stress and Its Clinical Implications
Abstract
Oxidative stress plays a crucial role in the pathophysiology of numerous diseases, including cardiovascular disorders, diabetes, neurodegenerative conditions, and chronic kidney disease. Reactive oxygen species (ROS) and reactive nitrogen species (RNS) are key contributors to cellular damage through oxidative and nitrosative stress, affecting cellular processes such as apoptosis, inflammation, and fibrosis. This review explores the molecular mechanisms underlying oxidative stress, its connection to various diseases, and the role of enzymatic and non-enzymatic antioxidants in counteracting its harmful effects. Emerging therapeutic strategies, such as Nrf2 activators and mitochondria-targeted antioxidants, are highlighted as promising tools for disease prevention and treatment. Understanding the interplay between oxidative stress and disease progression can pave the way for precision medicine approaches, offering more personalized and effective treatments.
1. INTRODUCTION
A free radical is any molecule or atom that possesses unpaired electrons or has an unpaired electron in its outer shell. The usefulness or toxicity of a substance in biological systems will be influenced by its location, production rate, the presence of nearby free radicals, and the molecules it subsequently interacts with. The utility of free radicals as mediators is established since they oversee numerous processes such as vascular tone regulation, which in turn affects blood pressure, and they may also be involved in the intermediate metabolism of biological substances [1]. The lifespan of the unstable radicals and their effects is quite brief, typically lasting from a few seconds to 10 −9 s. The half-life of certain radicals is influenced by the surrounding environment. For example, consider the half-life of NO•; in an oxygen-saturated solution, it persists for only a few minutes, similar to stabilized radicals, as most free radicals are short-lived and rapidly undergo chemical reactions (Fig. 1).
1.1. ROS (Reactive Oxygen Species)
ROS (Fig. 2) represents the largest group of free radicals in living organisms, significantly influencing cellular functions such as proliferation, differentiation, and migration, as well as apoptosis and necrosis. Therefore, they can potentially serve as anticancer agents. It is a continuous cycle of their creation and operation. Moderate to low amounts of ROS and RNS are essential for maintaining several important physiological functions, such as redox homeostasis and regulating key transcription factors [2].
1.2. Oxidative Stress
According to the definition given by the International Cancer Society, oxidative stress is a condition that can occur when there is an overabundance of unstable molecules called free radicals in the body, along with an inadequate supply of antioxidants to counteract them. This imbalance will ultimately lead to different levels of harm to cells and tissues. Numerous factors can contribute to oxidative stress, including obesity, unhealthy diets, smoking, alcohol consumption, use of specific medications or hallucinogens, and exposure to environmental elements like radiation, toxins, air pollution, pesticides, and sunlight. Aging, along with the onset of chronic inflammation, cancer, and several other unfortunate illnesses, will result from prolonged oxidative stress. Numerous disorders significantly impact oxygen metabolism in the human body, such as hypoxia, oxidative stress, nitrosative stress, endoplasmic reticulum stress (ERS), mitochondrial dysfunction, and carbonyl stress.
1.3. Nitrosative Stress
Nitrosative stress (Fig. 3) is consistently linked to oxidative stress. Reactive oxygen species (ROS), including superoxide anion (O-2), singlet oxygen (1O2), hydroxyl radical (OH), hydrogen peroxide (H2O2), peroxynitrite anion (ONOO-), and nitric oxide (NO), are crucial in oxidative stress and engage with one another as well as with the pathways responsible for the generation and elimination of reactive nitrogen species (RNS), leading to mutual regulation. ONOO- generated can nitrate several biomolecules, such as proteins, lipids, and DNA, resulting in the formation of 3-nitro-tyrosine (3-NT) [3-5].
The primary feature of nitrosative stress is the nitrosylation of tyrosine. Protein nitrosation is a significant alteration in nitrosative stress, a post-translational modification resulting from RNA/ROS interactions. Recent research indicates that 3-NT production can act as a specific biomarker for nitrosative stress, offering a dependable method to track intracellular nitric oxide (ONOO-) generation and localization, as well as the extent of cell death. Nitrosative stress may induce the nitrosation of tyrosine in proteins, resulting in lipid peroxidation, DNA strand breakage, damage to cell membranes, inactivation of enzymes, and the triggering of cell death signaling pathways. Nitrosative stress impacts disease via these essential biological mechanisms, influencing processes like cell signaling, mitochondrial energy metabolism, mRNA transcription, protein post-translational modifications, and ion functionality [6-8].
1.3.1. Nitrosative Stress in COVID-19
There is considerable research investigating the effects of nitrosative stress in people infected with COVID-19. For example, in a comparison of nitrosative stress markers between SARS-CoV-2-infected patients, recovered individuals, and healthy controls, it was observed that nitric oxide (NO), S-nitrosothiols and nitrotyrosine (NT) levels were significantly elevated in COVID-19 patients compared to controls. Throughout COVID-19, excessive production of reactive oxygen species (ROS) and reactive nitrogen species (RNS) is observed, leading to oxidative and nitrosative stress. In the human body, nitric oxide (NO), a key reactive nitrogen species (RNS), is produced by L-arginine through a process facilitated by the nitric oxide synthase (NOS) family of enzymes, which includes neuronal NOS (nNOS), endothelial NOS (eNOS) and inducible NOS (iNOS). These enzymes are found in infiltrating inflammatory cells. Under typical physiological conditions, NOS provides advantageous functions, including relaxation of vascular and bronchial smooth muscle, prevention of platelet accumulation, and participation in neurotransmission and immune responses. Nevertheless, an overproduction of NO can lead to proinflammatory impacts, resulting in bronchial hyperreactivity and damage to pulmonary endothelium, both of which have been noted in patients with COVID-19 [9-11].
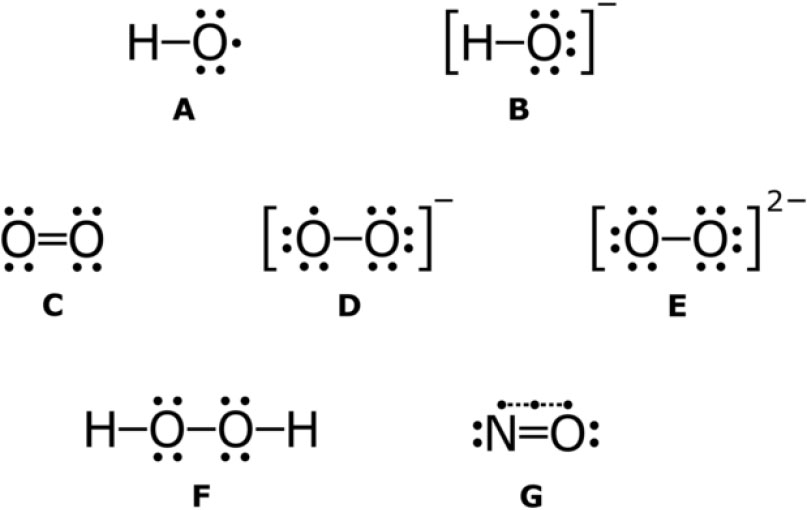
Some examples of reactive oxygen species with Lewis structures. A: hydroxyl radical B: hydroxide ion C: singlet oxygen D: superoxide anion E: peroxide ion F: hydrogen peroxide G: nitric oxide (nitrogen monoxide).
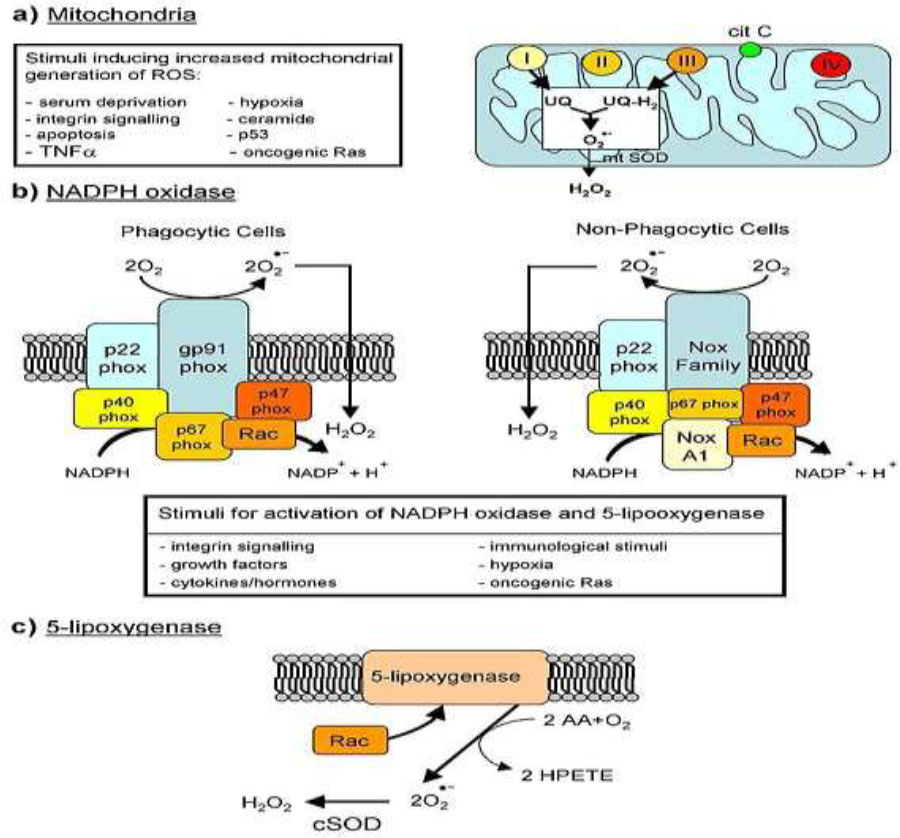
Major cellular sources of reactive oxygen species in living cells.
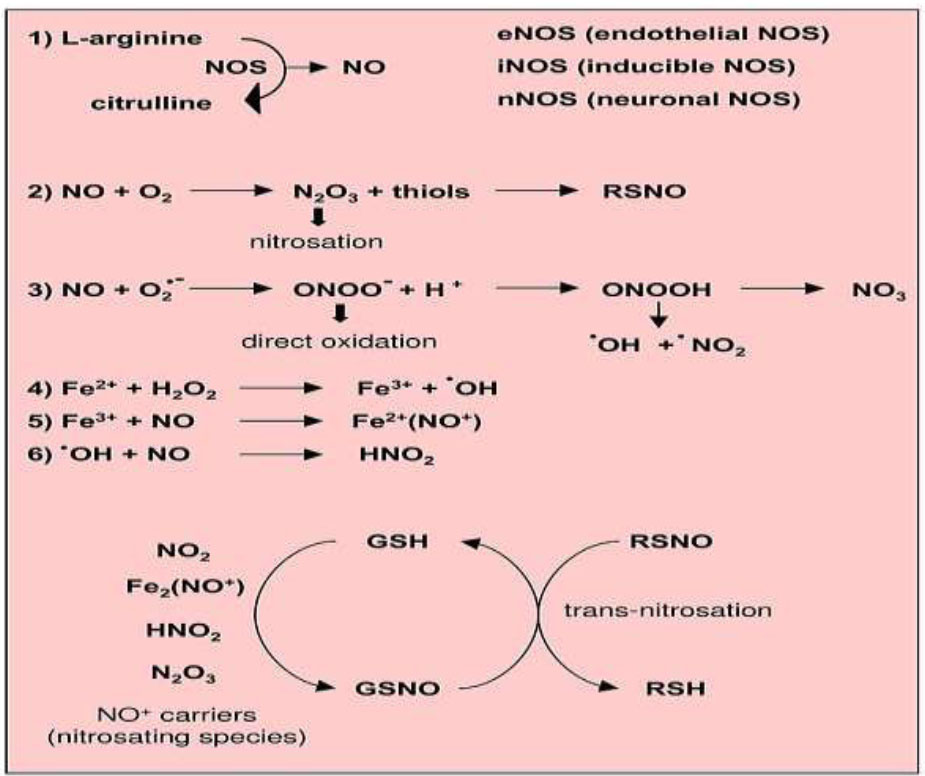
Reactions leading to generation of nitric oxide and reactive nitrogen species.
In the host organism, the infection process initiates when the spike protein (S) of SARS-CoV-2 attaches to the ACE2 receptors and transmembrane serine protease 2 (TMPRSS2). This binding aids the virus's penetration into endothelial cells, initiating the attachment of angiotensin II to the angiotensin II type 1 receptor (AT-1R). This activation results in the stimulation of nicotinamide adenine dinucleotide phosphate oxidase (NADPH oxidase), which subsequently enhances the production of reactive oxygen species (ROS). The generation of ROS triggers multiple signaling pathways that aid in the production of proinflammatory interleukins. These cytokines decrease the presence of endogenous nitric oxide (NO), prostaglandins, and their derivatives by forwarding endothelial dysfunction and injury. Moreover, heightened concentrations of proinflammatory markers and prothrombotic factors raise the chances of serious chronic complications, impacting not just the respiratory system but also the vascular and nervous systems. In the present research, NO concentrations were observed to be greater in COVID-19 patients than in the control group. The first stage of infection entails the attachment of the SARS-CoV-2 spike protein to TMPRSS2 (Transmembrane protease, serine 2 is an enzyme that in humans is encoded by the TMPRSS2 gene) and Angiotensin-converting enzyme 2 (ACE2), resulting in the infection of endothelial cells, which causes angiotensin II to bind to Angiotensin II receptor type 1 (AT-1R), activate NADPH oxidase, and elevate ROS production [12-15].
2. FREE RADICALS AND ANTIOXIDANT ACTIVITY
Biological systems must regulate the interactions and side effects of the vast array of free radicals, whether oxidized or reduced, that are either beneficial or detrimental to the organism's functions. There exists an internal mechanism that is generally successful in managing oxidative side effects, although it may not always be effective. The enzymatic pathway associated with oxidation can be classified into enzymatic antioxidants and non-enzymatic antioxidants. The key antioxidant enzymes that play a direct role in neutralizing free radicals are superoxide dismutase (SOD), catalase (CAT), glutathione peroxidase (GPx), and glutathione reductase (GRx). Glutathione reductases (GR, GLR, GSH- or GSSG-reductases) are proteins that depend on the adenine flavin dinucleotide (FAD) and are ubiquitous like GSH itself. The role of SOD, serving as the primary defense against free radicals, involves the catalytic conversion of anionic peroxide (O2-) into hydrogen peroxide (H2O2) through reduction. The hydrogen peroxide produced from the reaction is transformed into water and oxygen (O2) by catalase (CAT) or glutathione peroxidase (GPx). GPx, a selenium-containing enzyme, detoxifies H2O2 by employing reduced glutathione (GSH), converting it to oxidized glutathione (GSSG). Glutathione reductase (GRx), an enzyme classified as a flavoprotein, converts GSSG back to GSH by utilizing NADPH for its reducing power. Along with hydrogen peroxide, GPx also diminishes lipid and non-peroxide substances while oxidizing glutathione (GSH) during this process [16].
Non-enzymatic antioxidants are categorized into dietary and metabolic antioxidants. Metabolic antioxidants, categorized as endogenous antioxidants, are generated by metabolic processes within the body and consist of substances like lipoic acid, glutathione, L-arginine, coenzyme Q10, melatonin, uric acid, bilirubin, and metal-binding proteins like transferrin. In comparison, dietary antioxidants are external, as they cannot be synthesized by the body and need to be acquired from food or supplements. These consist of vitamins E and C, carotenoids, trace minerals (like selenium, manganese, and zinc), flavonoids, and omega-3 and omega-6 fatty acids. Lack of dietary antioxidants can play a role in the onset of numerous chronic and degenerative diseases, given that each nutrient possesses a distinct structure and antioxidant function [17].
Unregulated generation of free radicals may result in significant health issues. Free radicals may harm proteins, resulting in alterations to their structure and a reduction in enzyme activity. Damage to DNA caused by oxidation results in various types of oxidative DNA harm, which can lead to mutations. The body possesses mechanisms to combat these attacks through the utilization of DNA repair enzymes and/or antioxidant substances. In the absence of adequate regulation, oxidative stress may lead to numerous chronic and degenerative diseases, contribute to the aging process, and trigger specific acute medical issues like trauma or stroke [18, 19].
Several of the most active antioxidant enzymes include heme oxygenase, reductase, thioredoxin, glutathione-S-transferase (GST), GPx, CAT and SOD. These enzymes are essential for the conversion of toxic substances into safe products. Peroxides produced during metabolic activities are removed by GPx and GST. GRd is crucial for maintaining the balance between oxidized glutathione (GSSG) and reduced glutathione (GSH), as the GSSG/GSH ratio serves as a reliable measure of oxidative stress. Therefore, GRd works by increasing the levels of GSH, which is crucial for maintaining the reduction-oxidant balance in living organisms. Although GPx is present in every cell, CAT is mainly found in peroxisomes. The brain is particularly vulnerable to free radical damage because the concentration of GPx is seven times that of CAT. The largest amount of CAT is present in red blood cells, kidneys, and liver, where the majority of hydrogen peroxide is broken down [20]. Another common illustration of the extent of damage that free radical activity can cause is the adverse effects of nitric oxide (NO) reactions. Although NO is not particularly reactive, the formation of harmful, reactive types of NO is not inevitable. Its cytotoxic effects are mainly attributed to peroxynitrite, which is formed through the diffusion-controlled reaction between NO and peroxide anion. Peroxynitrite locks to lipids, proteins, and DNA through coordinated oxidative forms or through reverse radical-activated components. These reactions lead to cellular responses that vary from small, innocuous changes in cell signaling to extreme oxidative damage, resulting in cellular damage or apoptosis. Within the body, the peroxynitrite plays an imperative neurotrophic role in diseases such as stroke, dead myocardial tissue, persistent cardiac frustration, diabetes, circulatory anesthesia, unremitting inflammatory disorders, cancer, and neurodegenerative conditions [21].
An incredibly effective method to avoid the cytotoxic impact of free radicals is through prevention. The significant presence of lipids within the cellular system, along with their roles, emphasizes the importance of comprehending the effects and processes of lipid peroxidation in biological systems. Polyunsaturated fatty acids (PUFAs) serve as substrates for lipid peroxidation because their molecule contains active methylenes. The bonds between hydrogen and carbon in the methylene groups possess very low bond energy, making it easier for the hydrogen atom to be eliminated in radical reactions. It has been established that alpha-tocopherol is the most effective antioxidant to safeguard membranes from oxidation by interacting with different lipid radicals generated in the lipid peroxidation process. Antioxidant compounds like t-butylhydroxyanisole (BHA), t-butylhydroxytoluene (BHT), and vitamin E prevent lipid peroxidation caused by the Fe2+/ascorbic acid complex in rat liver microsomes [22, 23]. In cases of DNA damage caused by free radicals, antioxidants consist of both external and internal sources that can be ingested through food to meet the body's natural requirements. These agents help cleanse the body of free radicals and stop additional damage. Fractionated components of both natural and modified radicals of Rhaponticum carthamoides exhibit DNA repair capabilities and antioxidant defense against oxidative stress-induced DNA damage in Chinese hamster ovary (CHO) cells. Methane components from plants such as Tamarind indica, Adhatoda vasica, Centella asiatica, Pseudomugii furcatus, and Kocuria indica contribute to protection against DNA damage, while substances such as trans-resveratrol and p-cumaric acid in the ethanolic fraction of pistachio shoots exhibit protective effects against oxidative DNA damage [24, 25].
These substances can neutralize free radicals and help protect macromolecules such as DNA and proteins from damage. However, according to the available evidence and analysis of many studies in this area, the effects of specific antioxidant compounds on the prevention of DNA damage are still being debated. For some antioxidants, their protective benefits vary with dosage, suggesting that the right amount is essential. The administration of these compounds in incorrect doses may not only be ineffective in preventing DNA damage but may also exacerbate DNA fragmentation. A similar concern relates to their function in reducing or preventing cancer risk. For example, antioxidants that stop the growth of procarcinogens from their precursors, including carbon, could help prevent the formation of nitroso compounds [26], or inhibitors that prevent compounds in the cancer envelope from engaging the cellular target, such as flavones, isothiocyanates, and phenols. In addition, blocking agents can be classified into three main categories: those that limit carcinogen activation to the active carcinogenic state, those that stimulate an enzyme system that can detoxify carcinogens, and those that can interact with cancer-inducing agents to stop them from affecting cellular targets [26, 27]. Suppressive agents work by limiting carcinogenesis by inhibiting its processes, with examples of protease inhibitors, sea salt, and retinoic acid [28]. The interesting results suggest that fruit and vegetable consumption can help shield humans from harmful oxidation. These foods serve as excellent sources of antioxidants, which have been shown to be effective in aiding the management of cardiovascular disease. In addition, the antioxidants present in fruits and vegetables could help prevent neurodegenerative disorders such as Parkinson's disease and Alzheimer's disease. An overabundance of reactive species is often linked to medical issues such as ulcers, depression, cardiovascular disease, and rheumatoid arthritis. It is thought that antioxidants are essential for the prevention of these diseases. In addition, antioxidants have been shown to be beneficial in treating problems related to sexual development, male infertility, and kidney stones [29].
3. FREE RADICALS AND DISEASES
A comprehensive account of all disease cases linked to the effects of free radicals is unfeasible, as it pertains not only to present instances but also to those that will be documented in the future. Nevertheless, their participation in the onset or advancement of numerous pathological diseases is well established.
3.1. Oxidative Stress, Diabetes and Cardiovascular Function
Increased oxidative stress speeds up the creation of advanced glycation end products (AGEs), which are a complex blend of cross-linked proteins with modified structures. In conditions such as diabetes, obesity, Alzheimer's disease (AD), various neurological disorders, atherosclerosis, and amyotrophic lateral sclerosis, elevated levels of AGEs have been observed. As a result, AGEs, such as N-carboxymethyl lysine (CML), serve as markers of disease advancement. By interacting with the receptor for advanced glycation end products (RAGE), AGEs trigger a cascade of detrimental signaling pathways that result in the generation of proinflammatory cytokines. This mechanism escalates oxidative stress, leading to a self-reinforcing cycle of harm [30].
Inhibitors of AGEs, AGE modulators, and RAGE antagonists represent hopeful treatment alternatives for numerous conditions, such as diabetes and Alzheimer's disease (AD). The intricate nature of AGEs and the absence of thoroughly validated, rigorously tested processes for their creation pose difficulties in creating effective therapies aimed at oxidative stress and aging-related conditions. AGEs are primarily found in amyloid beta (Ab) plaques and neurofibrillary tangles (NFTs) in Alzheimer's disease. Furthermore, the relationship between AGEs and RAGE (binding of AGE to RAGE) plays a role in the development of pancreatic cancers, particularly in hyperglycemic conditions common in diabetes and obesity. AGE-RAGE interaction and the oxidative stress it triggers are crucial elements in the progression of diabetic kidney disease. Diabetes significantly contributes to the development of Alzheimer's disease (AD), with the two conditions exhibiting shared biomarkers, including increased levels of AGEs and other oxidative stress indicators like 4-hydroxy-trans-2-nonenal (HNE), which is a lipid peroxidation byproduct. Reactive oxygen species (ROS) related to cardiovascular work play a double role. In restricted amounts, ROS within the cardiovascular framework has illustrated positive impacts, such as anti-atherosclerotic characteristics, improving angiogenesis, and advertising endogenous cardio security. In any case, when ROS levels rise excessively, they cause cellular damage, as oxidative stress contributes to the onset and/or progression of cardiovascular diseases such as endothelial dysfunction, atherosclerosis, myocardial ischemia/reperfusion injury, heart failure, and arrhythmias [31-34].
In cardiovascular infection, oxidative stretch regularly changes the expression of quality. Increased levels of ROS will certainly decrease the activity of translational components, particularly NF-κB, activation protein-1 (AP-1), and the family of peroxisome proliferator-activated receptor (PPAR) family [35]. Furthermore, due to the increased ROS season, one of the initiating events of atherogenesis, along with other cardiovascular diseases associated with endothelial rupture, is an oxidative modification of low-density lipoprotein (LDL) [36]. In fact, both cell membranes and LDL, high in phospholipids, are very insensitive and, therefore, helpless to oxidative modifications. These conditions often result in the disabling of redox-sensitive signaling pathways. Oxidized phospholipids will navigate to pathways represented by or dependent on receptors, thus having the ability to activate endothelial cells. This can initiate an arrangement of reactions that will be negative for life forms as they drive the expression of endothelial handle atoms, attract monocytes, initiate endothelial cytotoxic effects, and improve proinflammatory quality action in addition to cell growth components. All these components lead to endothelial brokenness, platelet conglomeration, metalloproteinase expression, and advanced thrombogenesis.
3.2. Oxidative Stress in Aging
The scientific community has consistently focused on understanding the causes of aging. Harman describes aging as the gradual buildup of different detrimental changes in cells and tissues over time, which elevates the likelihood of disease and mortality. Nonetheless, despite a clear and thorough definition, as well as a thoroughly examined collection of traits, aging continues to be a difficult and elusive subject in biological research, primarily because of its inherently intricate and multifactorial characteristics. Furthermore, it can be challenging to differentiate between the impacts of typical aging and those caused by age-related illnesses. As a result, although disciplines such as physiology, genetics, epidemiology, and demography have created many theories supported by solid observational data to account for the aging process, none have succeeded completely. The exact mechanisms that elucidate the aging process in all species and systems are still not well understood and do not consistently offer the required insights [37, 38].
In the digestion system, subsequent research has shown that negligible levels of pro-oxidant atoms can affect the activation of translation components. In particular, a developing clustering of particles, such as different kinases, phosphatases, and translational variables from distinct signaling pathways, is accepted to be controlled by the intracellular redox state. By extension, some translational variables, such as the small GTP-binding protein Rac, are recognized to revitalize ROS-producing chemicals such as NADPH oxidase and the generation of ROS themselves, acting as controllers of individual reactivity. The effects of redox balance result in either activation or inactivation of translation variables based on the specific signaling pathways that are locked in. Maturation-related translation variables controlled by redox states include the oncogenic p53, Forkhead translation variables, activator protein-1 (AP-1), and NF-kB. The p53 protein, a tumor silencer, is essential for the control of cell cycle arrest, apoptosis, and cellular senescence. Forkhead translation elements, a critical family of proteins, are identified by a mitigated DNA-binding site referred to as the “Forkhead box.” These proteins are predominant in eukaryotic cells and, based on clinical evidence, play a significant part in overseeing cell cycle capture, stretch reactions, apoptosis, and life expectancy [39-41].
In the endogenous apoptotic pathway, studies have shown that proteins of the mitochondrial permeability transition pore complex, which controls mitochondrial membrane potential, are direct targets of reactive oxygen species (ROS). These proteins consist of the adenine nucleotide translocator located in the inner membrane, the age-related anion channel in the outer membrane, and cyclophilin D present in the mitochondrial matrix. Pro-oxidants that can affect mitochondrial membrane potential include not only compounds such as t-butyl hydroperoxide and diamide but also lipid peroxidation derivatives such as 4-hydroxy-2-nonenal. In addition, increasing evidence suggests that oxidative damage to organelles such as lysosomes and the endoplasmic reticulum can induce interactions with mitochondria, resulting in the initiation of apoptosis via an endogenous signaling pathway. Recent studies on the redox protein p66Shc may provide an understanding of the relationship between oxidative stress-induced apoptosis and biological aging. The redox protein p66Shc represents the third isoform found in the Shc family of proteins, which were first identified as signal transduction adaptors involved in mitogenic signaling through Ras, a small GTP-binding protein. Recent data indicate that p66Shc functions as an unusual signal transducer that can be affected by oxidative stress and is also involved in hydrogen peroxide (H2O2) production. Although p66Shc-deficient mice live 30% longer than controls, p66Shc-deficient cells derived from knockout mice show resistance to ROS-induced apoptosis. Numerous studies suggest that p66Shc could affect the mitochondrial permeability transition pore, contributing significantly to oxidative stress-induced apoptosis [42-50].
3.3. Oxidative Stress in Autophagy
Autophagy (Fig. 4) involves the encapsulation of large proteins, membrane pieces, and organelles into autophagic vesicles, which are then transported to and broken down in lysosomes. Recent interest has been sparked by the initial genetic findings revealing that autophagy is crucial for extending the lifespan of the nematode Caenorhabditis elegans [51]. Targeted inhibition of autophagy through RNAi methods eliminated life extension in C. elegans with a mutated gene in the insulin-like signaling pathway. As a housekeeping mechanism for cells, autophagy plays a crucial role in breaking down and recycling damaged cellular elements to promote cell survival during stressful situations like nutrient scarcity [52]. Conversely, current evidence indicates that autophagy plays a direct role in cell death triggered by both cytokines and chemicals.
3.4. Oxidative Stress in Kidney Disease
Renal dysfunction is a global health challenge with considerable economic benefits. It is estimated that the annual treatment cost for a persistent patient with persistent kidney disease is about 20 thousand US dollars. Renal disorders are classified into two main types: acute kidney injury (AKI) and stable kidney disease (CKD). In AKI, progression occurs rapidly, controlled by a decrease in renal work, which occurs with the accumulation of harmful by-products of nitrogen and creatinine metabolism in the patient's circulatory system. CHD in all stages, which has an extended period of motion, has been recognized in a range of 7 to 12% of patients worldwide. The estimated incidence of CHD is generally 20-200 per million people, and the annual incidence of CHD is about 2 million people. Renal tubules contain several mitochondria, as the reabsorption of solutes requires remarkable vitality [53]. Free radicals and prooxidants produced at various stages of AKI and CKD movement can escalate damage, enhance inflammatory responses, and contribute to genuine complications in further organs regularly monitored in AKI and CKD, such as cardiovascular problems and neurological disorders [54, 55].
Various oxidative stress markers, measurable in serum or tissues, will assess the condition and are also useful for monitoring disease progression [56]. The most widespread cause of AKI is hypopressure, resulting from reduced blood flow to the kidneys. In addition, ischemia is often observed as well. Ischemia and reperfusion are also triggers of oxidative stress, with mitochondria serving as a major source of ROS in this context. In contrast, increasing evidence identifies oxidative stress as a critical factor in tubular interstitial fibrosis due to myofibroblast activation, in addition to glomerulosclerosis driven by mesenchymal sclerosis, podocyte abnormalities, and parietal epithelial cell damage [57]. In case of ischemic injury, the generation of reactive oxygen species such as hydroxyl radical, peroxynitrite, and perchloric acid is guaranteed. At the same time, antioxidant enzymes such as superoxide dismutase (SOD), catalase and glutathione reductase are
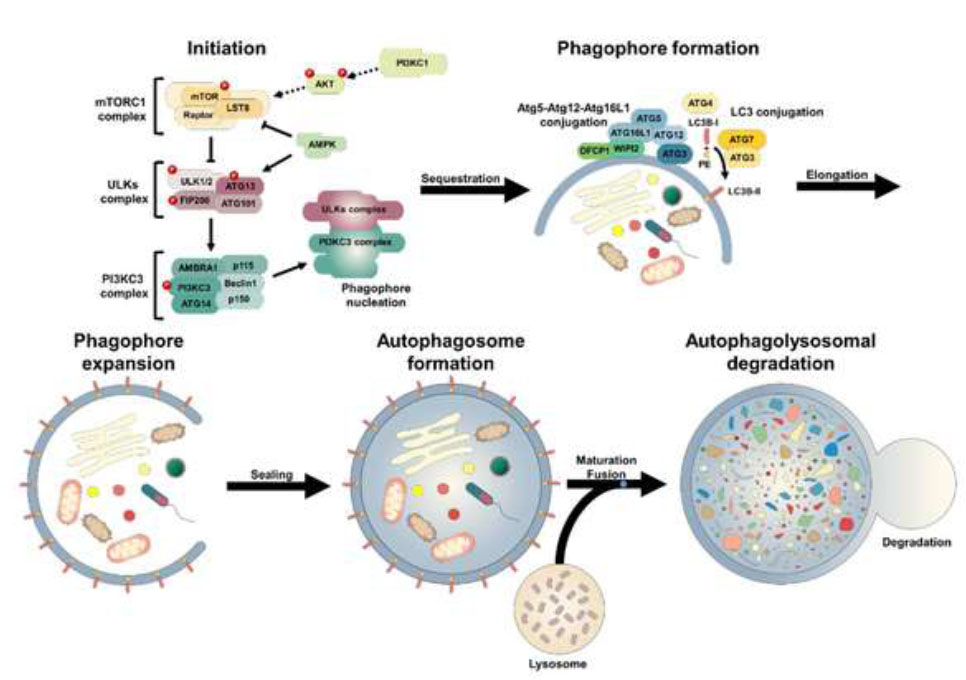
Autophagy process. Available online under the terms of the Creative Commons Attribution Non-Commercial License 4.0.
reduced. This has been discovered in renal tissue after renal ischemia and nephrotoxicity [58]. The triggering cycle leads to ischemic damage while enhancing the expression of proinflammatory cytokines and activating phagocytes that also produce ROS. Consequently, ROS affects vasoconstriction and resistance of renal blood vessels [59].
3.5. Recent In Vivo Studies
Recent research highlights the role of oxidative stress in diseases, particularly focusing on cardiovascular, kidney, diabetes, and neurodegenerative diseases. The effect of antioxidants, such as coenzyme Q10 (CoQ10), flavonoids, carotenoids, and resveratrol in reducing the incidence and progression of CVD has been studied. CoQ10 and carotenoids inhibit lipid peroxidation processes, thereby exerting a protective influence on the cardiac muscle [60, 61]. Clinical trials evaluating anti-oxidant supplements have failed to improve atherosclerosis, but new ROS scavengers specifically targeting mitochondrial ROS, statins, and renin-angiotensin system inhibitors exert antioxidative effects [62, 63]. Another study shows that mitochondria-mediated targeting may protect against ROS-mediated kidney injury in AKI to CKD progression [64], while vitamin C can lower renal injury and oxidative damage [64, 65]. Several clinical and preclinical studies have shown that AGEs mediation plays an important role in reducing and preventing vascular complications of diabetes. This can be achieved not only by proper blood glucose control but also through diet and medical intervention [66]. The effectiveness of curcumin and vitamin E in reducing oxidative stress in diabetic animals has been investigated, emphasizing the therapeutic potential for diabetic complications [67]. Lifestyle factors, such as exercise and diet, have been shown to reduce oxidative stress levels and exhibit neuroprotective effects [68]. Phytochemical antioxidant compounds also act as potential therapeutic agents in AD disease with a limitation of low bioavailability due to their poor water solubility [69]. Personalized treatment plans for patients with breast cancer combining endocrine therapy drugs combining with ROS modulation therapy could also be a novel treatment [70].
3.6. Innovative Therapeutic Strategies
3.6.1. Newer Mechanisms of Action of Antioxidants (Nrf2 activators, mitochondria-targeted antioxidants)
3.6.1.1. Nrf2 Activators
Nrf2 (Nuclear factor erythroid 2-related factor 2) is a transcription factor that regulates the expression of genes responsible for antioxidant defense and detoxification. Under normal conditions, Nrf2 remains bound to the cytoplasm by the protein Keap1. When cells are exposed to oxidative stress, Nrf2 is released, which then translocates to the nucleus and activates the expression of protective genes. Nrf2 activators are targeted to inhibit the Nrf2-Keap1 interaction, thereby enhancing cellular defense against oxidative stress [71, 72]. Agents with protective effects against oxidative stress of vascular endothelial cells mainly include phenylpropanoids, flavonoids, terpenoids, and alkaloids. The mechanisms by which they act are related to the activation of Nrf2/HO-1 signalling, a finding promising for the development of new drugs for the treatment of atherosclerosis and other cardiovascular diseases [73].
3.6.1.2. Mitochondria-targeted Antioxidants
Mitochondria are major sources of ROS production due to their role in cellular respiration. The accumulation of ROS in mitochondria can lead to their dysfunction and cellular damage. Mitochondria-targeted antioxidants are designed to selectively accumulate in mitochondria, neutralising ROS directly at their source. Novel mitochondria-targeted antioxidant compounds have been studied, in vitro and in vivo, to counteract oxidative stress in mitochondria associated with neurodegenerative diseases such as Alzheimer's, Parkinson's, and amyotrophic lateral sclerosis [74]. The therapeutic potential has been demonstrated for MitoQ, MitoVitE and MitoTEMPO. MitoQ is a derivative of plastoquinone (Plastoquinone), linked to a positively charged substance (triphenylphosphonium - TPP+). It targets mitochondria due to its electrochemical membrane potential. MitoVitE is a synthetic form of vitamin E (tocopherol) bound to triphenylphosphonium (TPP+). Vitamin E is known for its antioxidant properties, and targeting mitochondria increases its effectiveness in cellular organelles affected by oxidative stress. MitoVitE protects mitochondria from lipid oxidation and DNA damage. MitoTEMPO is an antioxidant derivative of TEMPO (tetramethylpiperidine nitroxyl oxide), linked to triphenylphosphonium (TPP+). It has the ability to neutralize oxygen free radicals (ROS) and reduce oxidative stress in mitochondria [75-77].
3.6.2. Precision Medicine
Precision Medicine aims to personalise the therapeutic approach, taking into account the unique genetic and molecular characteristics of each patient. In this context, the use of antioxidant therapies can be tailored based on specific gene profiles and biomarkers, enhancing the efficacy and safety of interventions. Understanding the genetic variants that affect oxidative stress and the body's antioxidant capacity is critical for the development of targeted therapies. Genes encoding enzymes such as superoxide dismutase, catalase, and glutathione-S-transferase play an important role in antioxidant defense. Polymorphisms in these genes may affect enzyme activity, making some individuals more susceptible to oxidative damage. The identification of these genetic variants through molecular diagnostic methods allows the categorisation of patients into subgroups with different needs. For example, individuals with reduced SOD activity may benefit from supplements that enhance the function of this enzyme. In addition, measuring levels of specific biomarkers, such as oxidized LDL, malondialdehyde and isoprostanes, can provide information on the degree of oxidative stress and guide the dosage and duration of antioxidant therapy. The integration of genetic information and biomarkers into clinical practice can improve the prognosis and treatment of various conditions associated with oxidative stress, such as cardiovascular disease, neurodegenerative disorders and cancer. Monitoring programmes combining genetic analyses with regular biomarker assessment can contribute to early detection and personalised intervention [78, 79].
Protein Misfolding Cyclic Amplification (PMCA) technology allows highly sensitive detection of prions, contributing to early diagnosis and in-depth study of protein misfolding mechanisms leading to neurodegenerative disorders. This technology has proven valuable for understanding the complexity of prion diseases and developing new diagnostic and therapeutic approaches. One of the main technologies used for highly sensitive detection of conventional infectious agents (SARS-CoV-2, HIV, hepatitis) is PCR nucleic acid amplification. Prions are composed of a protein responsible for a group of fatal neurodegenerative diseases. Similar to the detection of viruses by PCR through the amplification of viral genetic material, prions can be detected by PMCA through the amplification of the infectious protein. This allowed the detection of tiny amounts of prions in various body fluids and peripheral tissues, even from individuals with a pre-symptomatic condition. The 100% sensitivity and specificity demonstrated by PMCA in blood studies make them a diagnostic tool for prion diseases [79, 80]. Recent studies suggest that proteins such as β-amyloid (Aβ) and tau in Alzheimer's disease, as well as α-synuclein in Parkinson's disease, can adopt prionic properties, leading to self-induced accumulation and spreading of pathological proteins. The importance of understanding sawtooth mechanisms in a wider range of neurodegenerative disorders paves the way for new diagnostic and therapeutic approaches [81].
3.6.3. How can Future Studies Improve our Understanding of Oxidative Stress in Disease Progression?
Future studies may improve our understanding of oxidative stress in disease progression through various approaches such as:
1. Development of sensitive and specific biomarkers that can detect oxidative stress early in the disease process. The discovery of new drug targets will often focus on proteins, transcription factors, and enzymes due to their critical role in many cellular processes and pathological conditions.
2. Investigation of molecular mechanisms and studies of interactions through which oxidative stress contributes to cell damage, apoptosis and inflammation.
3. Analysis of the role of epigenetic modifications associated with oxidative stress.
4. Studies on the use of nanotechnology in the directed delivery of antioxidant agents.
5. Analysis of the interaction between oxidative stress and lifestyle factors (diet, exercise).
6. Monitoring patients to investigate the relationship between oxidative stress and the progression of chronic diseases such as cancer, diabetes, and neurodegenerative diseases.
7. Evaluate the effectiveness of new treatments in clinical trials.
8. Use of advanced technologies (genomics, proteomics, metabolomics) to uncover new pathways involved in oxidative stress.
9. Application of artificial intelligence and machine learning to model and predict disease progression based on oxidative stress.
CONCLUSION
Free radicals function as mediators, influencing various processes, including the regulation of vascular tone and blood pressure, as well as participating in intermediate metabolism. ROS represent the largest group of free radicals within living organisms, significantly influencing cellular functions. They play an essential role in processes such as cell proliferation, differentiation, apoptosis, and necrosis and can serve as anti-cancer agents. Oxidative stress is defined as a situation that arises when there is an excess of unstable molecules known as free radicals in the body, combined with an insufficient amount of antioxidants to eliminate them. This is bound to cause harm to cells and tissues to a greater or lesser extent. When numerous elements can cause oxidative stress, they encompass reasons such as obesity, unhealthy eating, smoking, alcohol consumption, the use of specific drugs or hallucinogens, and exposure to environmental influences like radiation, toxins, air pollution, pesticides, and sunlight. Aging, along with the onset of chronic inflammation, cancer, and several other undesirable illnesses, will result from prolonged oxidative stress. Nitrosative stress is consistently linked with oxidative stress. Reactive oxygen species, including superoxide anion, singlet oxygen, hydroxyl radical, hydrogen peroxide, peroxynitrite anion, and nitric oxide, contribute to oxidative stress, interact amongst themselves, and with the mechanisms for the generation and elimination of reactive nitrogen species, leading to a reciprocal regulation. ONOO-generated can nitrate diverse biomolecules such as proteins, lipids, and DNA, resulting in the formation of 3-nitro-tyrosine. Biological systems must regulate the interactions and side effects of a vast array of free radicals, whether oxidized or reduced, that may be beneficial or detrimental to the organism's functions. An internal mechanism is typically efficient in managing oxidative side effects, though it may not always be. We can classify the enzymatic pathway associated with oxidation into enzymatic antioxidants and non-enzymatic antioxidants. The escalating oxidative stress results in a higher rate of advanced glycosylation end product formation, which is a complicated blend of altered proteins. Elevated levels of AGEs are seen in diabetes, obesity, Alzheimer's disease, other neurological conditions, atherosclerosis, and amyotrophic lateral sclerosis. In cardiovascular diseases, oxidative stress typically modifies gene expression. Elevated levels of ROS diminish the function of transcription factors, especially NF-κB, activation protein-1, and the peroxisome proliferator-activated receptor family. Ischemia and lower pressure from decreased blood flow to the kidneys provoke oxidative stress, with mitochondria serving as the primary source of ROS in this context. On the other hand, oxidative stress is crucial in tubular interstitial fibrosis due to myofibroblast activation, and it also contributes to glomerulosclerosis resulting from microvascular cell stiffening, podocyte dysplasia, and damage to parietal epithelial cells. By integrating the roles of oxidative and nitrosative stress in a broad spectrum of diseases (cardiovascular, kidney, and metabolic), as well as focusing on molecular mechanisms like mitochondrial dysfunction, fibrosis, and AGEs formation, this study addresses gaps in the understanding of how oxidative stress underpins various disease processes.
However, the complexity of oxidative stress and its dual role in cellular signaling and pathology complicates the development of effective therapies. Recent advancements in targeted antioxidant therapies, such as mitochondria-specific agents and Nrf2 activators, offer promising prospects for combating oxidative stress-related diseases. Future research should focus on identifying novel biomarkers, understanding the genetic predisposition to oxidative damage, and exploring precision medicine strategies to optimize antioxidant-based interventions. By shedding light on these mechanisms, new diagnostic and therapeutic avenues may emerge, enhancing disease prevention and patient outcomes.
AUTHORS’ CONTRIBUTIONS
It is hereby acknowledged that all authors have accepted responsibility for the manuscript's content and consented to its submission. They have meticulously reviewed all results and unanimously approved the final version of the manuscript.
LIST OF ABBREVIATIONS
RNS | = Reactive Nitrogen Species |
ROS | = Reactive Oxygen Species |
ERS | = Endoplasmic Reticulum Stress |
SOP | = Super-oxide Dismutas |
CAT | = Catalase |
GPx | = Glutathione Per-oxidase |
GRx | = Glutathione Reductase |
PMCA | = Protein Misfolding Cyclic Amplification |
ACKNOWLEDGEMENTS
Declared none.