All published articles of this journal are available on ScienceDirect.
Study on the Interaction of 4'-Hydroxychalcones and their Mannich Derivatives with Calf Thymus DNA by TLC and Spectroscopic Methods, a DNA Cleavage Study
Abstract
Background:
Phenolic Mannich bases derived from hydroxychalcones show remarkable cytotoxic potencies towards cancer cell lines. However, the exact mechanism of action is still partially uncleared.
Objective:
Interaction of two hydroxychalcones and their Mannich derivatives with calf thymus DNA (ctDNA) has been investigated.
Methods:
Thin-layer chromatography and UV-Vis spectroscopic method were used for studying the interaction. The binding constant has been determined by UV-Vis spectrophotometric titration. The DNA cleavage activity of the compounds was studied by agarose gel electrophoresis.
Results:
Interaction of the compounds with ctDNA exhibited relatively high intrinsic binding constant (4-5x104 M-1). The results indicate existence of weak, non-covalent interactions between the investigated derivatives with ctDNA. Some compounds showed a slight DNA cleavage activity with pBR322.
Conclusion:
The obtained results provide additional knowledge on the previously documented cytotoxicity against tumor cell lines of the hydroxychalcones and their Mannich-derivatives.
1. INTRODUCTION
Chalcones (1,3-diphenyl-prop-2-en-1-ones), are intermediary compounds of the biosynthetic pathway of a very large and widespread group of plant constituents, known collectively as flavonoids [1]. Among the naturally occurring chalcones and their synthetic analogs, several compounds displayed cytotoxic, antitumor, anti-inflammatory, and chemo preventive properties, which are well documented in the literature [1-7].
It was concluded that biological activity, including the cytotoxic effect of chalcones and their some cyclic derivatives, might be partially a consequence of non-covalent interaction between the compounds and cellular macromolecules (proteins, DNA) [8-11]. Since DNA is an important cellular receptor, many compounds exert their tumor cytotoxic effect through binding to DNA, thereby influencing the cell-cycle, changing its replication, inhibiting cell growth, blocking the division, and resulting in apoptosis or cell death. There are primarily three different ways by which anticancer drugs interact with DNA: (a) through control of transcription factors and polymerases, (b) through RNA binding to DNA double helix to form nucleic acid triple helix structures and (c) through binding of small molecules to DNA double-helical structures [12]. Small molecules can react with DNA via covalent and non-covalent binding, with interest generally focusing on the latter. The non-covalent binding of small molecules to DNA involves binding on the outside of the helix (external binding) through electrostatic interaction, intercalation between base pairs, and binding in the minor or major DNA grooves [13-15].
Mannich bases are beta-aminoketones, which are generated from various substrates through the introduction of an aminomethyl function utilizing the Mannich reaction. Aminoalkylation can change lipophilicity of a drug leading to an improved delivery in the human body [16]. The solubility of the Mannich bases could be further increased as a result of their protonation to form the respective ammonium salt [17]. Mannich bases are an important group of compounds in medicinal chemistry; they display a wide range of biological activities [18]. It was shown that the most effective compounds against tumor cells are Mannich derivatives of acetophenones and structurally related α,β-unsaturated ketones, which exert their cytotoxic action through the alkylation of cellular thiols such as glutathione or cysteine [18, 19].
Investigation of Mannich derivatives of chalcones has received much attention in recent years. Several Mannich bases derived from hydroxychalcones show remarkable cytotoxic potencies towards cancer cell lines [20-25]. The studies showed that the 4-hydroxy group in the A ring and heterocyclic rings in the B ring contribute to the bioactivity of the Mannich bases [25]. Some Mannich derivatives of chalcones present potent anti-inflammatory and antioxidant activities [26]. Mannich bases of heterocyclic chalcones inhibited nitric oxide production in lipopolysaccharide- and interferon-γ-stimulated RAW 264.7 macrophages [27]. Mannich bases derivatives of flawokawain B display potent activities against the acetylcholinesterase enzyme [28, 29]. A novel Mannich base derivative might be a potential multifunctional agent for the treatment of Alzheimer's disease and offered a starting point for the design of new multitarget AChE/MAO-B inhibitors based on the chalcone scaffold [30].
Previous investigations showed 4-chloro-4'-hydroxychalcone (Fig. 1 (2A)) as a potent inhibitor (IC50 of 3.8 μM) of MDA-MB-231 (estrogen receptor-negative) cells, commonly used to model late-stage breast cancer [31]. Further, the GSH activity of the compound was considered to play a role in the mechanism of action of the molecule. However, it is proved that the Mannich derivative of the compound (Fig. 1 (2B)) showed higher spontaneous GSH reactivity than the parent 4'-hydoxychalcone (2A) [32].
As a continuation of the previous works [32], here we report on synthesis and DNA binding properties of two 4'-hydroxychalcones (1A and 2A) and their Mannich derivatives (Fig. 1 (1B and 2B)). The selection was made based on the previous results of 2A, showing promising antiproliferative activity against estrogen receptor-negative MDA-MB-231 cells [31]. Furthermore, 4-alkoxychalcones have proven to be potent antiproliferative chalcone derivatives [1-6]. For better understanding the molecular mechanism of cytotoxic effects of 4'-hydroxychalcones and their Mannich analogs, here, we report results of thin-layer chromatographic and UV-Vis studies on the interaction of two 4'-hydroxychalcones (1A, 2A) and their Mannich derivatives (Fig. 1 (1B, 2B)) with calf thymus DNA (ctDNA). The effect of DNA-binder phenolic anticancer drugs can be associated with their DNS-cleavage activity [15]. Based on these previous observations, the DNA cleavage capacity of the compounds was also investigated.
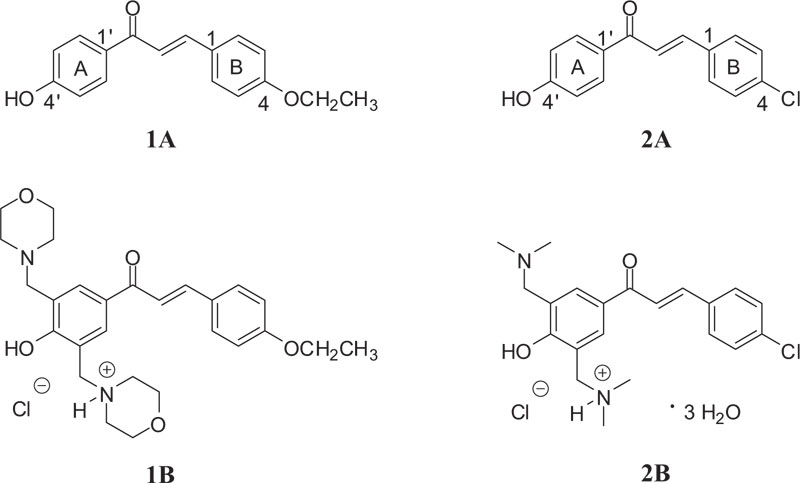
2. MATERIALS AND METHODS
2.1. Materials
All chemicals used were of the analytical grade available and, if otherwise not specified, purchased from Sigma-Aldrich Hungary (Budapest, Hungary).
Infrared (I.R.) spectra were performed on a Bruker IFS-55 FT spectrometer (Bruker Optik GmbH, Leipzig, Germany). 1H and 13C NMR spectra were recorded on a Bruker Avance III 500 (500.15/125.77 MHz for 1H/13C) spectrometer (Bruker Optik GmbH, Ettlingen, Germany). The melting points were measured on a Barnstead Electrothermal 9100 melting point apparatus (San Francisco, USA). The mass spectrometry analyses were carried out in Q-Tof III micro from Bruker Daltonics equipment (Bruker Optik GmbH, Ettlingen, Germany).
2.2. Synthesis of Compounds
4'-Hydroxychalcones 1A and 2A, as well as their bis Mannich derivatives 1B and 2B (Fig. 1), were synthesized in the Institute of Chemistry, Federal University of Goiás, Goiânia-GO, Brazil.
Hydroxychalcone 1A was synthesized by Claisen-Schmidt condensation, as previously described by Kumar et al. [33], with slight modifications. Equimolar amounts of 4-hydroxyacetophenone (10 mmol) and 4-ethoxybenzaldehyde (10 mmol) were added to 15 mL of 40% NaOH methanolic solution. The reaction mixture was stirred at 64 ºC for 12 h when Thin-Layer Chromatography (TLC) indicated completion of the reaction. Then, cold water (5 mL) was added to the media and acidified with 10% hydrochloric acid until pH 3. The resulting yellow solid was crystallized from methanol to obtain the expected chalcone 1A in 75% yield. The structure of compound 1A was confirmed based on the melting point measurement and spectroscopic methods by comparison with literature data [33, 34].
(2E)-1-(4-hydroxyphenyl)-3-(4-ethoxyphenyl)prop-2-en-1-one (1A): yield.: 75%; m.p.: 187-190 ºC; IR (KBr) λ/cm-1 3420 (OH-Ar), 1610 (C=O), 1603 (Ar-CO-C=C-Ar), 1573 (OCH2CH3), 1347 (OCH2CH3); 1H NMR (500 MHz, DMSO-d6) δ 8.05 (d, 2H, J 8.91), 7.80 (d, 2H, J 8.69) 7.75 (d, 1H, J 15.48, Hβ), 7.65 (d, 1H, J 15.48, Hα), 6.99 (d, 2H, J 8.69), 6.89 (d, 2H, J 8.91), 4.10 (q, 2H, J 6.99, OCH2CH3), 1.35 (t, 3H, J 6.99, OCH2CH3);13C NMR (126 MHz, DMSO-d6) δ 187.53 (C=O), 162.45, 160.89, 143.16 (Cβ), 131.47, 129.84, 127.86, 120.00 (Cα), 115.78, 115.24, 63.78 (OCH2CH3), 15.02 (OCH2CH3). IR, 1H NMR and 13C NMR spectra of the compound are shown in Figs. (1S-4S).
The bis Mannich chalcone 1B was synthesized from the corresponding hydroxychalcone 1A for the first time by following the classical conditions of the Mannich reaction for phenolic compounds [35]. First, morpholine (2.4 mmol) in ethanol (15 mL) was heated under reflux with formaldehyde (3.0 mmol) for 2 h. Then, hydroxychalcone 1A (1.2 mmol) and few drops of 37% hydrochloric acid (0.15 mL) were added to the reaction medium and stirred for 96 h. The solution was concentrated, followed by the addition of a hydrogen chloride solution in dry diethyl ether to form the corresponding hydrochloride salt.
(2E)-1-[4-hydroxy-3,5-bis[(morfolin-4-ylmethyl) phenyl ]-3-(4-ethoxyphenyl)prop-2-en-1-one hydrated (1B): yield.: 39%; m.p.: 205-209 ºC; IR (KBr) λ/cm-1 3120 (O-H), 2973 (N-H), 1647 (C=O), 1600 (Ar-CO-C=C-Ar), 1250 (OCH2CH3), 1045 (OCH2CH3); 1H NMR (500 MHz, DMSO-d6) δ 8.53 (s, 2H), 8.04 (d, 1H, J 15.72, Hβ), 7.92 (d, 2H, J 8.65), 7.72 (d, 1H, J 15.72, Hα), 6.99 (d, 2H, J 8.65), 4.55 (s, 4H), 4,10 (q, 2H, OCH2CH3), 3.90 (m, 8H), 3.28 (m, 8H), 1,35 (t, 3H, OCH2CH3); 13C NMR (126 MHz, DMSO-d6) δ 192.40 (C=O), 166.08, 148.92, 141.46 (Cβ), 136.44, 136.36, 135.14, 132.72, 125.05, 123.27 (Cα), 120.19, 68.78, 68.63 (OCH2CH3), 59.37, 56.17, 19.98 (OCH2CH3). HRMS: calculated for C27H34N2O5 [MH+]: 466.2540, measured 466.2607. IR, 1H NMR, 13C NMR, and HRMS spectra of the compound are shown in Figs. (5S-9S).
Synthesis and structural characterization of investigated chalcone 2A and derivative 2B have been previously described [32]. Their purity of each investigated sample was checked by TLC.
2.3. Investigation of DNA Binding by Thin-layer Chromatography (TLC)
Thin-layer chromatographic investigations of the DNA binding affinity of the compounds were performed using silanized (reversed-phase; R.P.) silica gel 60F254 (0.25 mm) plates (Merck, Germany, No. 5747). The TLC plates were pre-developed with methanol/50 mM sodium hydrogen phosphate (pH 7.4) mixture (8:2 v/v). First, the tested compounds (1.6 mM in methanol) were incubated with ctDNA (1 mg/ml in 50 mM sodium hydrogen phosphate, pH 7.4 (equal to 1.6 mM base pairs)) (1:1 v/v) for 60 min at 37 °C, and the incubated solution was spotted at the origin. Secondly, tested compounds (1.6 mM in methanol) were spotted at the origin, followed by spotting of ctDNA (1 mg/ml in 50 mM sodium hydrogen phosphate, pH 7.4 (equal to 1.6 mM base pairs)) at the same positions. Ethidium bromide (E.B.) was used as a positive control. The plates were developed with a methanol/50 mM sodium hydrogen phosphate (pH 7.4) mixture (8:2 v/v). The location of ctDNA was visualized by spraying the plate with anisaldehyde, which gives blue color with DNA. Investigated compounds were visualized by U.V. illumination.
2.4. Investigation of DNA Binding by UV-Vis Spectrophotometry
UV-Vis measurements were performed on a Jasco V-670 spectrophotometer (Japan) using 1 cm path length quartz cuvettes at ambient temperature. For DNA binding studies, a stock solution of ctDNA (0.5 mg/ml) was prepared by dissolving ctDNA in tris(hydroxymethyl)aminomethane hydrochloride (Tris-HCl) buffer (10 mM, pH 7.4) overnight at 5 °C and stirred several times to ensure the homogeneity of the solution. The purity of DNA was determined by measuring the ratio A260/A280 (> 1.8) [36]. The concentration of the ctDNA solution (expressed as base pairs) was determined from the U.V. absorbance at 260 nm using the molar extinction coefficient value at this wavelength (ε = 6600 1/mol*cm) [37]. The investigated compounds (1A, 1B and 2A, 2B) were dissolved in dimethyl sulphoxide (DMSO) immediately before used. The freshly prepared solutions were diluted with 10 mM Tris-HCl solution (pH 7.4) to the final concentrations (0 – 20 μM). The concentration of DMSO in the mixtures was 1% v/v. Measurements were performed in the presence of ctDNA (50 μM) after 2 min equilibration at room temperature in the dark. Alteration of absorbance by time was followed for 30 minutes in a solution containing chalcone (20 μM) and ctDNA (50 μM). Variation in the absorption spectrum of ctDNA (50 μM) has been studied as a function of chalcone concentration from 0 to 20 μM.
2.5. DNA Cleavage Study
The cleavage experiments of supercoiled pBR322 DNA (purchased from ThermoFisher Scientific, Biocenter Kft., Szeged, Hungary) (300 ng) by the investigated compounds (20 μM) in Tris-HCl (5 mM)/NaCl (50 mM) buffer (pH 7.2) were carried out using agarose gel electrophoresis [38, 39]. The samples were incubated at 37°C for 1 h. Then, a loading dye was added, and electrophoresis was carried out at 70 V for 90 min in TAE (tris-acetate-EDTA) buffer (40 mM Tris-acetate, 1 mM EDTA, pH 8.0) using 1% agarose gel containing 0.5 μg/ml ethidium bromide. After electrophoresis, bands were visualized under a U.V. illuminator. The cleavage efficiency was studied by the ability of the compounds to convert the supercoiled DNA (Form I) to a nicked circular form (Form II) and linear form (Form III).
3. RESULTS AND DISCUSSION
Several methods have been developed for investigation of the interaction of small molecules with DNA [12, 40]. To obtain information of the mechanism of action, binding behavior of compounds 1A, 1B and 2A, 2B (Fig. 1) with calf thymus DNA was studied on the molecular level in vitro using TLC and UV-Vis spectrophotometric methods.
The principle of the thin-layer chromatographic method is based on the ability of DNA to migrate on RP-18 F254 TLC plates, pre-developed with methanol/sodium hydrogen phosphate (50 mM; pH 7.4) mixture (8:2 v/v) as an elution system [41-43]. Free DNA moves along the plate and can be detected as a blue spot on RP-18 TLC after spraying with anisaldehyde reagent. In the presence of DNA intercalators (e.g., E.B.), a portion of DNA is bound as a complex, which remains at the origin or migrates only in a very short distance when the methanol/sodium hydrogen phosphate (50 mM; pH 7.4) mixture (8:2 v/v) is used as the mobile phase. In contrast, non-bounding test compounds allow DNA to leave the origin and move along the plate. The result revealed that compounds 1A, 1B, 2A, 2B showed binding affinity toward DNA compared to the known intercalator, ethidium bromide used as a reference (Fig. 2).
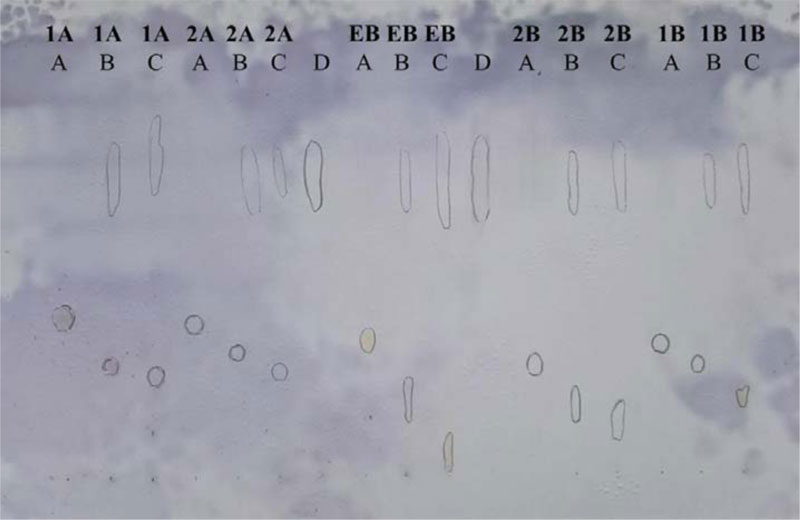
UV-Vis absorption spectroscopy is a simple and most commonly used instrumental method for studying the interaction of DNA with small molecules as ligands. Due to the interaction of DNA base pairs with the compounds, hypo- or hyperchromism or/and red or blue shifts in the UV-Vis spectrum of the compounds can be observed. The magnitude of the change in absorbance or the shift of the absorption peak is correlated with the strength of the interaction [12, 44]. Fig. (3) shows the absorption spectra of the free ctDNA, the free chalcones, and the ctDNA-chalcone mixtures. The absorption maxima of the mixture of compound 1B and DNA exhibited a weak hypsochromic (blue) shift relative to the absorption maxima of the free ligand (Table 1).
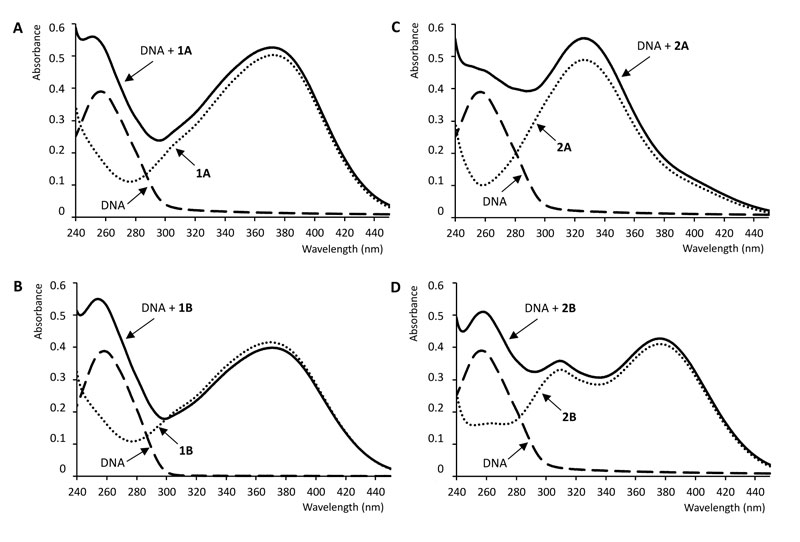
Compound |
λmax freea (nm) |
λmax bounda (nm) |
Δλ (nm) |
Binding constant (M-1) |
---|---|---|---|---|
1A | 352 | 352 251 |
0 7b |
6.1 x 104 |
2A | 327 | 327 258 |
0 0 |
- |
1B | 373 | 370 252 |
3 6b |
3.9 x 104 |
2B | 376 310 |
376 310 258 |
0 0 0 |
- |
bDifference of wavelength from the UV-Vis absorption maxima of the free ctDNA (λmax = 258 nm).
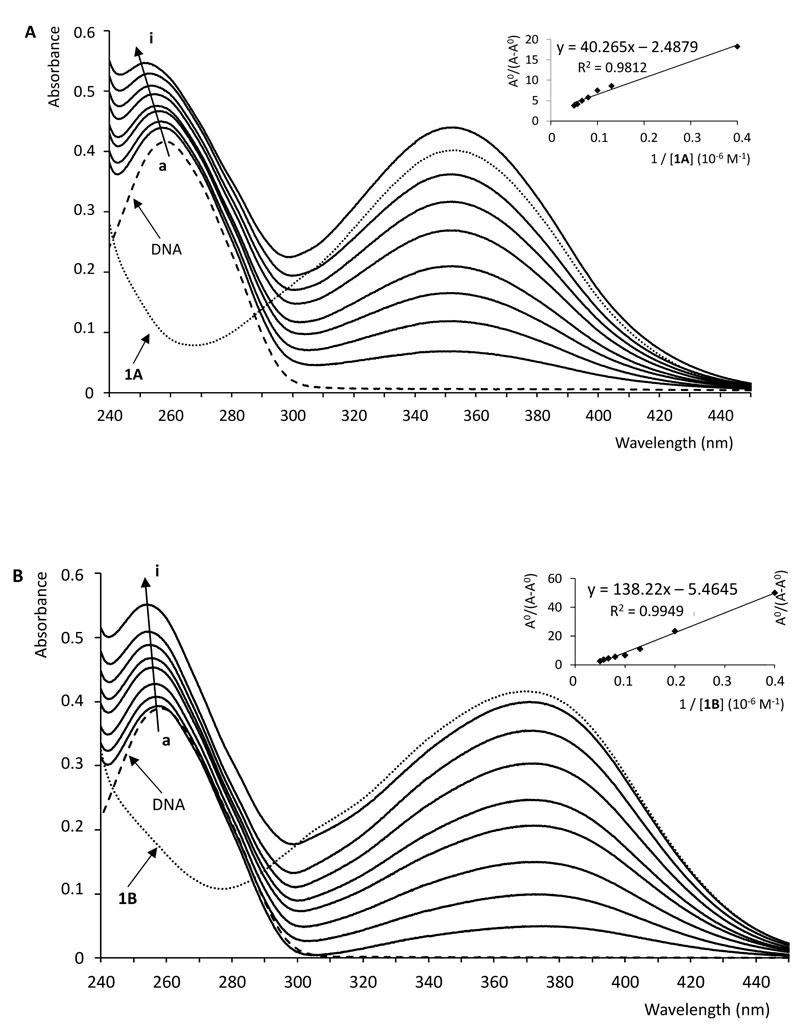
On the contrary, the presence of DNA did not cause a shift in the absorption maximum of 1A, 2A, and 2B. However, compound 1A and its Mannich-derivative (1B) caused a remarkable blue shift in the absorption maxima of ctDNA (Table 1). The intensity of absorption of 1B decreased on mixing with ctDNA. The measured absorbance values at the absorption maxima of the complexes did not change after the 2 min equilibration time.
With a fixed concentration of ctDNA, UV-Vis absorption spectra were recorded with the increasing amount of compounds 1A and 1B, respectively. As shown in Fig. (4), UV-Vis spectra displayed that the absorption of DNA (λmax = 258 nm) exhibited a proportional growth with the increasing concentration of the investigated chalcone. Meanwhile, the absorption value of the simple sum of the free ctDNA and the free 1B was a little bit higher than the measured value of the 1B-DNA complex. Accordingly, the interaction of 1B and DNA resulted in a weak hypochromic effect of the absorbance. On the other hand, in the case of compound 1A, a hyperchromic effect could be observed; the absorption value of the sum of the free DNA and the free 1A was lower than the measured value of the 1A-DNA complex.
Intercalation of small molecules into the DNA helix results in redshift (bathochromism) as well as hypochromism because of strong stacking interaction between an aromatic chromophore and the base pairs of the nucleic acid. Hypochromism arises from the contraction of the DNA in the helix axis as well as conformational changes [45]. Hyperchromism occurs as the result of possible non-covalent interactions, particularly electrostatic and groove binding between small molecules and ctDNA [12, 46-48]. Hyperchromism reflects the corresponding changes of DNA in its conformation after the small molecule is bound to it [45, 49]. The lack of any clear isosbestic point in the chalcone-ctDNA spectra refers to the fact that more than one type of binding modes may play a role in the overall binding, and/or the 1:1 chalcone:ctDNA stochiometry is not maintained during the process [44]. According to the observations, the investigated compounds show neither pure intercalator (redshift plus hypochromism) nor pure groove binding (high binding constant plus hyperchromism) characteristics, suggesting that non-covalent interactions play the major role in the interactions [46, 47, 50].
To compare the binding strength quantitatively, based on the variation in the absorption spectra of ctDNA upon binding to the chalcones, the Benesi-Hildebrand equation Eq. (1) can be applied to calculate the binding constant (K) [51, 52].
![]() |
(1) |
where A.O. and A represent the absorbance (λ =258 nm) of the ctDNA in the absence and presence of compounds 1A and 1B, respectively; εC and εD-C are the absorption coefficients of the chalcones (1A, 1B) and the chalcone-ctDNA complexes, respectively.
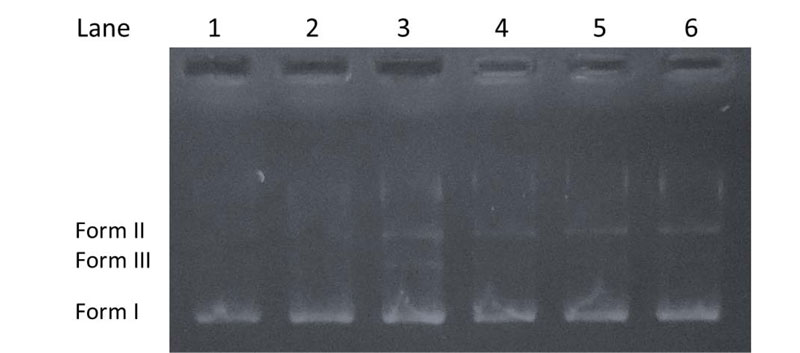
The plot of AO/(A-AO) versus 1/[chalcone (1A or 1B] was constructed by means of linear fitting of the absorption titration data (Fig. 4). The binding constants (K) were calculated from the ratio of the intercept to the slope. The binding constants of the interactions (Table 1) indicate that 1A and 1B have a relatively high affinity with the DNA double helix. The values are one or two orders of magnitude lower than those reported for intercalators [47, 50]. However, the obtained K values fit well with that of the well-established groove binding agent spermine [46]. Furthermore, the binding constant values are consistent with those reported for the interaction of some flavonoids and cyclic chalcone analogs with DNA [53, 54].
The capability of the chalcone derivatives to cleave DNA was tested using pBR322 plasmid, which might undergo transformation into relaxed circular form (Form II) from a supercoiled form (Form I) [47, 55]. When the circular plasmid DNA is subject to electrophoresis, a relatively fast migration is observed for the intact supercoiled Form I. When scission occurs in one of the strands (nicking), the supercoil relaxes to generate the open-circular Form II, which moves slower. If both strands are cleaved, a linear form (Form III) is formed, migrating between Form I and Form II [56]. Fig. (5) shows the cleavage results of pBR322 by the chalcone derivatives. It can be seen that no or very slight DNA cleavage was observed for control experiments (Lane 1-2) compared to the samples treated with the chalcones (Lane 3-6). The results indicate that each compound can induce a weak cleavage of the plasmid DNA.
CONCLUSION
The interaction of two hydroxychalcones and their Mannich derivatives with ctDNA was studied by TLC method and UV-Vis absorption spectroscopy. The binding reaction with the macromolecule was spontaneous, and presumably, non-covalent interaction played a significant role in the reaction. The observed differences in the spectral features suggest the importance of the electron-rich moieties of the compounds. The detected weak DNA cleavage activity confirms the previous results referring that interaction with DNA might be a contributing effect to the observed cytotoxicity. The obtained results provide additional knowledge on the pharmacological effect of hydroxychalcones and their Mannich derivatives.
ETHICS APPROVAL AND CONSENT TO PARTICIPATE
Not applicable.
HUMAN AND ANIMAL RIGHTS
Not applicable.
CONSENT FOR PUBLICATION
Not applicable.
AVAILABILITY OF DATA AND MATERIALS
Not applicable.
FUNDING
The study was supported by the EFOP Operational Program (Grant No. EFOP-3.6.1-16-2016-00004).
CONFLICT OF INTEREST
The author declares no conflict of interest, financial or otherwise.
ACKNOWLEDGEMENTS
Declared none.
SUPPLEMENTARY MATERIAL
Supplementary material is available on the publishers web site along with the published article.