All published articles of this journal are available on ScienceDirect.
Pharmacokinetics of a Mono-pyridinium-mono-aldoxime (K-347), a Potential Antidote in Organophosphate Poisoning
Abstract
Background:
Our recent work has been treating the pharmacokinetics of pyridinium aldoximes of various structures including their time-dependent distribution in the body of male rats and also the extent of blood-brain-barrier penetration.
Objective:
Our overall aim was to find a proper antidote in organophosphate poisoning with fast elimination.
Methods:
White male Wistar rats were intramuscularly injected with the aqueous solution of 3 µmol of K-347. The animals were sacrificed at different time periods following treatment; various tissues and body fluids were taken and homogenised. The level of K-347 was determined using reversed-phase HPLC. Dose-dependence of tissue level was also determined by using various doses, 3 µmol through 100 µmol of K-347.
Results:
The serum level of K-347 showed a definitely fast decline. K347 did not have any effect on Gram-positive and Gram-negative bacteria that we tested.
Conclusion:
The kinetics of K-347 showed an extremely fast offset, even in comparison with several other pyridinium aldoximes in clinical practice and in developmental stages.
1. INTRODUCTION
Tabun, sarin, etc. (phospho-organic compounds) are used by terrorists. Organophosphates are also applied as pesticides and insecticides. Proper handling and adequate use in agriculture may ensure safety, however, their side effects may be toxic in case of improper application.
The use of sarin by terrorists resulted in innocent human victims at the Tokyo metro [1] and recently in Syria [2]. It is important to underline that the rescue persons of a terrorist attack are in no less danger than the victims themselves. The use of pralidoxime and obidoxime only partially helps to restore acetylcholinesterase and butyrylcholinesterase activity. The therapy utilizes atropine, fluids, oxygen and either pralidoxime or obidoxime [3]. Pesticide handlers are subjected to depression to cholinesterases, both BuChE and AcChE [4].
Quantitative determination of pyridinium aldoxime-type cholinesterase reactivators can be done in various ways. HPLC is applied using UV absorbance [5], mass spectrometry [6] or electrochemical detection [7]. Sakurada et al. [8] discovered that pralidoxime can penetrate into the brain. Our work and results have also confirmed that both the brain and cerebrospinal fluid (a blood-free part of the central nervous system) contain a certain level of pyridinium aldoxime following its application [5].
Kuca and Musilek et al. [9-17] synthesized a large series of pyridinium aldoximes (PyrAlds). Our target was to study the pharmacokinetics of PyrAlds. The chemical structures of these compounds together with their lipophilicity (logP), and other characteristics are listed in Table 1.
Our recent work has been dealing with the pharmacokinetics of pyridinium aldoximes of various structures including their time-dependent distribution in the body of male rats and also the extent of blood-brain-barrier penetration. Chemically similar compounds were used, such as K117 and K127 [12]. We found that certain compounds with lipophilic substituents stayed in the sera and brain of rats for a long time.
Petroianu et al. [13] compared the ability of various pyridinium aldoximes to reactivate AcChE inhibited by paraoxon, and the classical ones (pralidoxime and HI-6 were found to be among the best reactivators), however, the effect of the novel aldoximes and their comparable reactivators proved to be similar. These compounds are presented in Table 1.
Compound | Chemical Structure | logP | TPSA (Å2) | MWcation | Ref. |
---|---|---|---|---|---|
K-347 |
![]() |
-1.89 | 36.47 | 213.28 | [14] |
K-027 |
![]() |
-7.82 | 85.47 | 286.37 | [15] |
K-048 |
![]() |
-7.63 | 83.47 | 300.40 | [16] |
K-117 |
![]() |
-7.77 | 82.17 | 316.40 | [17] |
K-127 |
![]() |
-8.47 | 92.67 | 316.40 | [17] |
K-203 |
![]() |
-7.88 | 83.44 | 298.38 | [18] |
K-269 |
![]() |
-3.47 | 104.31 | 329.42 | [14] |
K-456 |
![]() |
-7.82 | 83.44 | 286.37 | [14] |
K-867 |
![]() |
-6.78 | 83.44 | 332.82 | [15] |
K-868 |
![]() |
-7.23 | 83.44 | 355.25 | [15] |
K-870 |
![]() |
-6.58 | 83.44 | 367.26 | [15] |
Metoxime |
![]() |
-7.10 | 72.94 | 258.31 | [9] |
Obidoxime |
![]() |
-7,15 | 82,17 | 288.34 | [9] |
Pralidoxime |
![]() |
-3,36 | 36.47 | 137.18 | [3] |
A search for highly effective reactivators is an essential task; research on them is in progress [17-19]. Similarly, a short-stay and also a retarded-stay reactivator should be searched based on their in vivo pharmacokinetics. This paper was found to be successful in finding a pyridinium aldoxime compound with a short-term stay in the body.
2. MATERIALS AND METHODS
K-347 and K-870 were synthesized in the Department of Chemistry, University of Hradec Kralove; the chemical structures are given in Table 1. The reactivator effect of K-347 on tabun-intoxicated rats outlined by Karasova et al. [14].
Solvents and chemicals were purchased from commercial sources in the best possible quality, such as acetonitrile (LiChrosolv, gradient grade for liquid chromatography, Merck, Darmstadt), methanol (HPLC grade, Aldrich, Milwauke), 1-octane sulfonic acid, HPLC grade (Reanal, Budapest), citric acid hydrate, a.r., sodium acetate, a.r. and perchloric acid 70%, ACS grade (Molar Chemicals Kft., Halásztelek, Hungary).
2.1. Animals and Treatment
Rats (male, Wistar, 180-199 gram) were purchased from Toxicoop (Budapest, Hungary). Following a 24 h acclimatization period, rats were intramus- cularly injected with the freshly prepared aqueous solution of K-347. The solution was applied in one of the hindlimbs of rats in a volume of 0.2 mL. Rats were sacrificed 5, 15, 30, 60 and 120 min following administration. In the dose-dependence studies, 10, 20, 40, 70, 100 µmol doses of K347 were i.m. injected, and the animals were sacrificed at 30 min following the treatment. One of the 10 animals got lost after 10 minutes following its treatment with 70 µmol of K-347. Rats were anaesthetized and exsanguinated in the campus and serum was gained by centrifugation (1600 g, 15 min, 4 0C, Janetzky-K70, Berlin, Germany). CSF was collected by cisternal puncture through the foramen occipital magnum. All the other tissue samples were isolated immediately and kept at -80 ºC, not longer than 14 days. On the day of the analysis, the aliquots of the samples were homogenized in 0.3 M of perchloric acid (PCA) by an Ultra Turrax T25 Janke & Kunkel homogenizer (IKA Labortechnik, Staufen, Germany) at 20,000 rpm/min for 30 s at room temperature. Serum samples were homogenized with a 9-fold excess of PCA; 4-fold excess of PCA was added to all the other samples. The homogenates were centrifuged in an Eppendorf centrifuge (A. Hettich, Tuttlingen, Germany) at 14,000 rpm for 20 min at 4°C. The supernatants were directly used for HPLC.
The analytical equipment (JASCO 4000 series) contained the following modules: a quaternary gradient pump (PU-4180), a sample unit (AS-4050), and a photodiode array (PDA) detector (MD-4010). The analytical column was thermostated in an Antec Decade II electrochemical detector. The ChromNav 2.0 software was employed for instrument control and data acquisition (ABL&E-JASCO Hungary Ltd, Budapest, Hungary).
K-347 was assayed in isocratic chromatographic runs. A Phenomenex Kinetex EVO-C18 100x3 mm (particle size: 5 µm) column, thermostated at 40 °C, was used as the stationary phase. The mobile phase was a 80:10:10 ratio mixture of buffer solution:methanol:acetonitrile. The buffer solution contained citric acid (9.4 g/L), sodium acetate (10.8 g/L) and sodium octanesulfonate (3.7 g/L).
The injected sample volume was 20 µL, and the run time was 10 min. K-347 and the internal standard K-870 were detected at 300 nm. A 80:10:10 mixture of water, acetonitrile and methanol was used as flush solvent.
The peak area ratios of the analytes and those of the internal standard K-870 were used for evaluation. All the samples were measured in triplicates. Calibration was performed using 0.3 N aqueous perchloric acid solution containing an analyte and the internal standard between.0.234 and 46.9 µmol/L. The calibration model was linear with 1/x2 weights. Parameters of typical calibration curves for K347 are shown in Table 2.
The method was validated according to the effective guidelines of the European Medicines Agency and is shown in Table 2. The analysis was selective and no carry-over was observed.
Validation Parameter | Result |
---|---|
LOQ | 0.023 µmol/L |
LOD | 0.009 µmol/L |
Calibration (y = mx + b) | y=2.419-0.0355 |
R2 | 0.9982 |
Accuracy of back-calculated calibrator points (n=32) | 88.4-108.0% |
Accuracy (intra-day) | - |
level 1 (0.347 µmol/L) | 93.6% |
level 2 (0.591 µmol/L) | 99.4% |
level 3 (20.6 µmol/L) | 103% |
level 4 (44.1 µmol/L) | 106% |
Reproducibility (intra-day) | - |
level 1 (0.347 µmol/L) | 10.0% |
level 2 (0.591 µmol/L) | 5.4% |
level 3 (20.6 µmol/L) | 11.3% |
level 4 (44.1 µmol/L) | 3.7% |
Accuracy (inter-day) | - |
level 1 (0.347 µmol/L) | 99.0% |
level 2 (0.591 µmol/L) | 106% |
level 3 (20.6 µmol/L) | 88.1% |
level 4 (44.1 µmol/L) | 104% |
Reproducibility (inter-day) | - |
level 1 (0.347 µmol/L) | 10.4% |
level 2 (0.591 µmol/L) | 14.4% |
level 3 (20.6 µmol/L) | 3.9% |
level 4 (44.1 µmol/L) | 5.2% |
Freeze-thaw stability (3 cycles) | - |
level 2(0.591 µmol/L) | 102-115% |
level 4 (44.1 µmol/L) | 95.7-107% |
The robustness of the analytical method was evaluated in terms of the effect of buffer concentration, the concentration of the ion-pairing reagent and the content of the organic component in the eluent. A ±5% difference in buffer concentration caused a ±1.1% change in the retention time. The effect of a change in the concentration of the ion-pairing reagent was even smaller, with a ±5% concentration difference resulting in ±1.5% shift in the retention time. The retention of the analyte and that of the internal standard was, however, very sensitive to changes in the organic content of the mobile phase, decreasing the acetonitrile and methanol content from 10% to 8% in each shifted retention time of K-347 and K-870 from 6.7 to 4.1 min and from 8.0 min to 4.4 min, respectively. Employing a mobile phase containing 12% of acetonitrile and methanol, it increased the retention times to 12.5 min and 15.6 min, respectively. Because of the sensitivity of K-347 and K-870 to the organic content of the mobile phase, only the aqueous component was stored and was mixed with the employed organic solvents immediately before setting up the analytical system for each run.
2.2. Bacterial Strains
The following bacterial strains were used for the antibacterial susceptibility testing; Gram-positive bacteria: Bacillus subtilis ATCC6633, Methicillin sensitive Staphylococcus aureus ATCC29213, Enterococcus feacalis ATCC51299, and Gram-negative bacteria: Pseudomonas aeruginosa ATCC15442, Escherichia coli ATCC 25922, Klebsiella pneumoniae ATCC700603 (Table 3).
2.3. Antibacterial Susceptibility Testing
The efficacy of the prepared compounds was determined with the broth micro-dilution method according to the EUCAST guideline (www.eucast.org). Bacterial strains were grown on COS agar (Columbia agar + 5% sheep blood, Biomérieux, Budapest, Hungary) at 35.5 °C overnight. Appropriate numbers of colonies were suspended in physiological saline in order to reach a density of 0.5 McFarland for inoculation. Stock solutions containing the substances were prepared in distilled water and DMSO (1:1). These were two-fold serially diluted from 20 to 0.044 mg l−1 in cation-adjusted Mueller–Hinton broth (Biolab, Budapest, Hungary) and 100 μl of each dilution was transferred into microplate holes. Inoculation was carried out with 10 μl of each bacterial suspension. Incubation was performed at 35 °C for 24 h and minimal inhibitory concentrations (MICs) were determined visually.
3. RESULTS
Time-dependent changes of K-347 levels in rat serum and the brain are shown in Fig. (1).
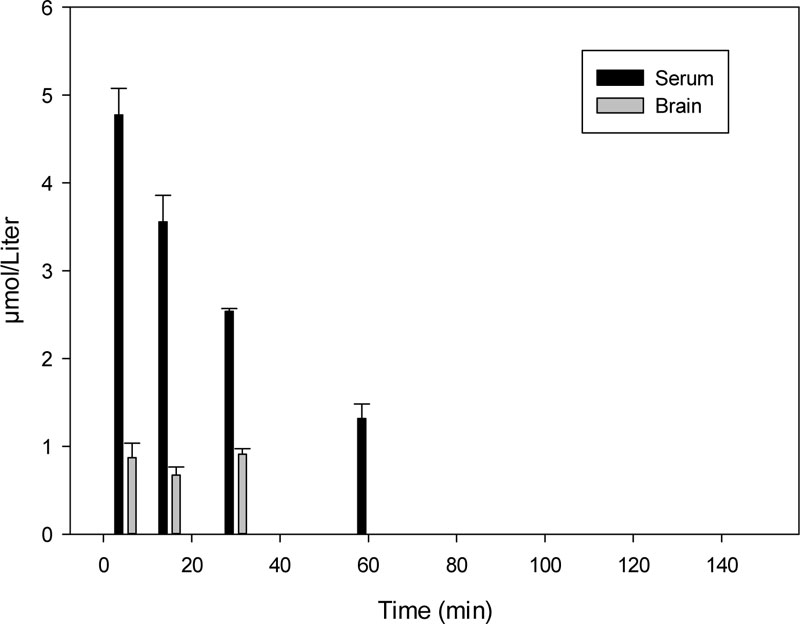
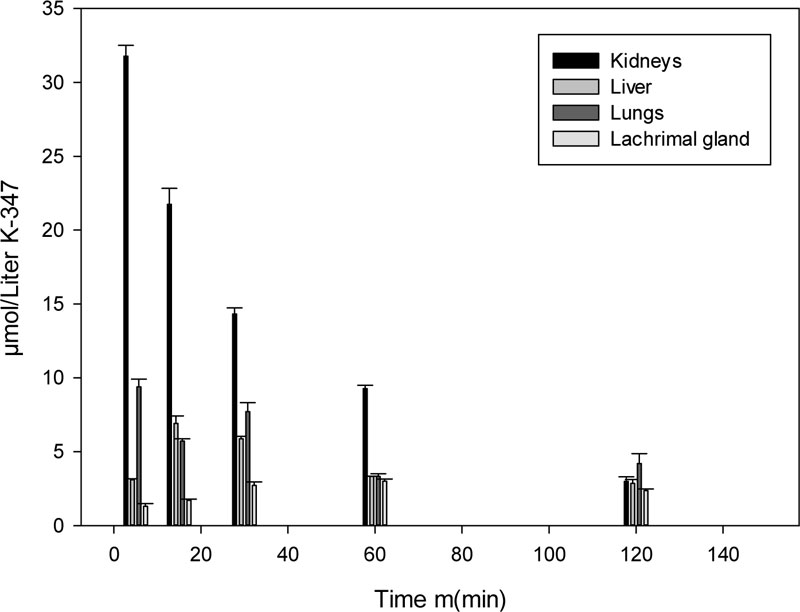
The 60- and 20-minute samples in the brain, and the 120-minute serum sample contained a K-347 level under the limit of determination (LOD) value following i.m. administration.
K-347 gets eliminated from the body of rats through the kidneys, lungs, livers and lacrimal glands. The essential organs of elimination are the kidneys, while the lungs also play a major role, as the level of K-347 in kidneys is continuously higher than that in the liver and the lacrimal glands (Fig. 2).
Dose-dependent pharmacokinetics shows proportional levels of K-347 in various tissues and cells. The serum level of K-347 was found to be increased with elevated doses of K-347 in the range of 10 to 100 µmol doses (Fig. 3).
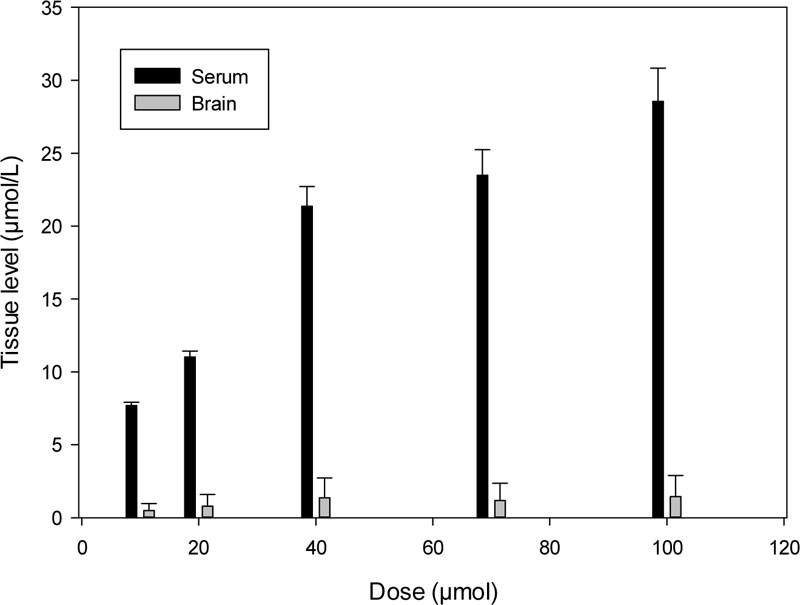
However, the K-347 content in the brain does not show a similar increase (Fig. 3). The extraction efficiency of the increased serum level was also increased, especially through the kidneys (Fig. 4).
The excretion of K-347 takes place through the kidneys, lungs, liver and lacrimal glands. Their dose-dependent K-347 levels are shown in Fig. (4).
The levels of K-347 in the testes and eyes were elevated with increasing doses as shown in Fig. (5).
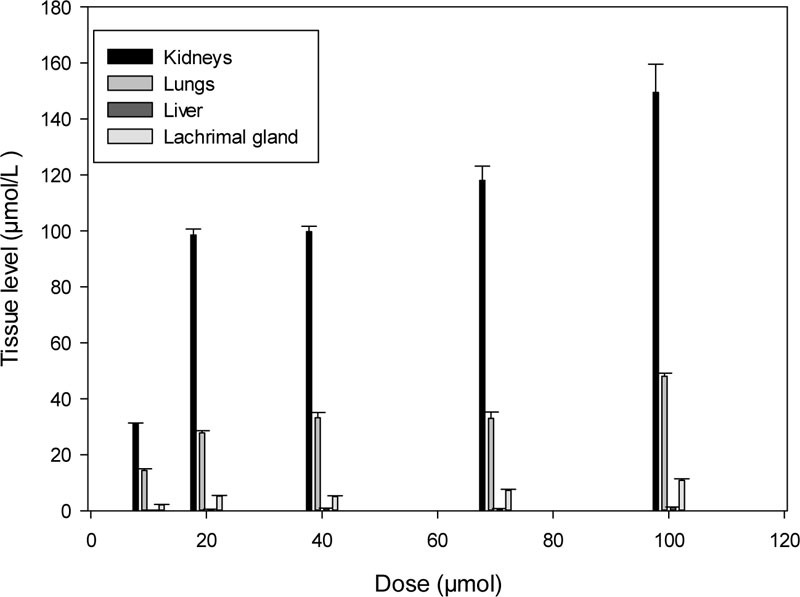
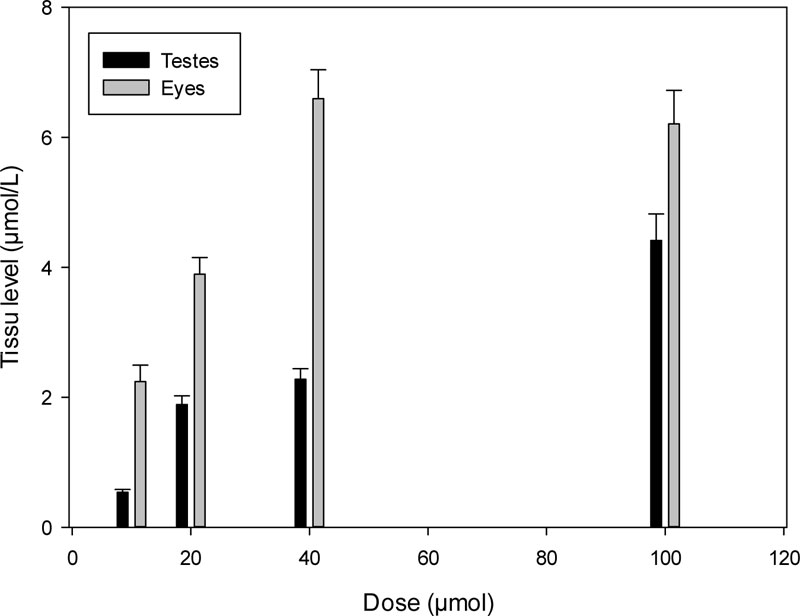
Agents | Bacillus subtilis ATCC6633 | Methicillin sensitive Staphylococcus aureus ATCC29213 | Enterococcus faecalis ATCC51299 | Pseudomonas aeruginosa ATCC15442 | Escherichia coli ATCC 25922 | Klebsiella pneumoniae ATCC700603 |
---|---|---|---|---|---|---|
K-27 | over 1400 | |||||
K-48 | over 1400 | |||||
K-117 | over 1400 | |||||
K-127 | over 1400 | |||||
K-269 | over 1400 | |||||
K-347 | over 1400 | |||||
K-867 | over 1400 | |||||
K-870 | over 1400 |
4. DISCUSSION
Xenobiotics can be eliminated from the body through the liver (either metabolized or non-metabolized), through the kidneys (by either glomerular filtration, tubular secretion or diffusion across the renal tubules) [20, 21]. Several, mainly minor elimination routes also exist, such as excretion through sweat, tears and saliva, which are generally nonessential [20, 21]. Elimination through the lungs is rarely mentioned, except when precise calculations of drug distribution are outlined [22]. Elimination of K-347 through the lungs is also less important than that through the kidneys, as its amount in the lungs is about half of its levels in the kidneys. However, the K-347 level in the lungs is higher than in the liver.
Chloride substitution on the pyridinium ring slows the rate of elimination and, therefore, the drug levels in the blood become higher. Effects of substitution of either aromatic or aliphatic carbohydrates to pyridinium aldoximes are even more pronounced. A long-lasting constant (or nearly constant) drug level can be detected in doses around 30 µmol/200 g in rats, where an alkyl chain, the substitution of an aromatic ring, or a condensed ring is coupled. The shape of the pharmacokinetic curve thereby transforms from a triangle-like shape into a trapezoid one. It can be the cause of limited glomerular fittration indicated by the increase in the molecular size of aldoxime.
The serum level of K-347 exhibits a monotonous decline. The fast pharmacokinetics of K-347 is probably caused by a possible intensive extraction, especially by glomerular filtration through the kidneys.
CONCLUSION
K-347 is a promising antidote to be used by rescue person(s) who help patients in organophosphate poisonings, especially when they should be in contact with the organophosphate-contaminated area for a short time. The fast decline of K-347 minimizes any potential side effects. Moreover, the absence of its antibacterial effect excludes any interference in later treatments with antibiotics.
ETHICS APPROVAL AND CONSENT TO PARTICIPATE
All the steps of animal handling, treatment procedures and sample preparations conformed to the 86/509/EEC regulation on the well-being of experimental animals and were performed according to the permission of the Local Committee of Animal Welfare Semmelweis University, Budapest, Hungary (Perm. No.: PE/EA/385-7/2018).
HUMAN AND ANIMAL RIGHTS
No humans were used. All the animal rights were fully considered according to the Animals Act 1986 Amendment Regulation (SI 2012/3039).
CONSENT FOR PUBLICATION
Not applicable.
AVAILABILITY OF DATA AND MATERIALS
Not applicable.
FUNDING
This project was financially sponsored by No. 126968 grant of the Hungarian National Office for Research and Development (Budapest, Hungary), by the Ministry of Education, Youth and Sports of the Czech Republic (No. 8F17004) and by the University of Hradec Kralove, Faculty of Sciences (Hradec Kralove, Czech Republic), No. VT2019-2021).
CONFLICT OF INTEREST
The authors declare no conflict of interest, financial or otherwise.
ACKNOWLEDGEMENTS
Pyridinium aldoximes were synthesized and supplied by the Department of Chemistry, Faculty of Sciences, University of Hradec Kralove, Czech Republic. Advice and cooperation by Mr. János Horváth, Mrs. Bogi Szalacsi, Mrs. Divikiné Gúth Györgyike, Ms. Krisztina Kecskés and Ms. Fanni Bákonyi are acknowledged.